- Search Menu
- Sign in through your institution
- Chemical Biology and Nucleic Acid Chemistry
- Computational Biology
- Critical Reviews and Perspectives
- Data Resources and Analyses
- Gene Regulation, Chromatin and Epigenetics
- Genome Integrity, Repair and Replication
- Methods Online
- Molecular Biology
- Nucleic Acid Enzymes
- RNA and RNA-protein complexes
- Structural Biology
- Synthetic Biology and Bioengineering
- Advance Articles
- Breakthrough Articles
- Special Collections
- Scope and Criteria for Consideration
- Author Guidelines
- Data Deposition Policy
- Database Issue Guidelines
- Web Server Issue Guidelines
- Submission Site
- About Nucleic Acids Research
- Editors & Editorial Board
- Information of Referees
- Self-Archiving Policy
- Dispatch Dates
- Advertising and Corporate Services
- Journals Career Network
- Journals on Oxford Academic
- Books on Oxford Academic

Advance articles
Distinct dna repair mechanisms prevent formaldehyde toxicity during development, reproduction and aging.

- View article
- Supplementary data
A new anti-CRISPR gene promotes the spread of drug-resistance plasmids in Klebsiella pneumoniae

A Plasmodium apicoplast-targeted unique exonuclease/FEN exhibits interspecies functional differences attributable to an insertion that alters DNA-binding

Correction to ‘N 2 -methylguanosine modifications on human tRNAs and snRNA U6 are important for cell proliferation, protein translation and pre-mRNA splicing’
Correction to ‘structure of an internal loop motif with three consecutive u·u mismatches from stem–loop 1 in the 3′-utr of the sars-cov-2 genomic rna’, correction to ‘overexpression of eif5 or its protein mimic 5mp perturbs eif2 function and induces atf4 translation through delayed re-initiation’, editorial: gary felsenfeld (1929–2024), the 22nd annual nucleic acids research web server issue 2024, enl reads histone β-hydroxybutyrylation to modulate gene transcription.

- Lay summary
Nanopores map the acid-base properties of a single site in a single DNA molecule

MicroRNA biogenesis is broadly disrupted by inhibition of the splicing factor SF3B1

Reinventing gene expression connectivity through regulatory and spatial structural empowerment via principal node aggregation graph neural network

ASH1L guards cis -regulatory elements against cyclobutane pyrimidine dimer induction

Enhancing insights into diseases through horizontal gene transfer event detection from gut microbiome

Phage-host co-evolution has led to distinct generalized transduction strategies

Motif-VI loop acts as a nucleotide valve in the West Nile Virus NS3 Helicase

RAD18 directs DNA double-strand break repair by homologous recombination to post-replicative chromatin

Binding of the TRF2 iDDR motif to RAD50 highlights a convergent evolutionary strategy to inactivate MRN at telomeres

Formation of left-handed helices by C2′-fluorinated nucleic acids under physiological salt conditions

Efficient activation of hundreds of LTR12C elements reveals cis -regulatory function determined by distinct epigenetic mechanisms

Cooperative Gsx2–DNA binding requires DNA bending and a novel Gsx2 homeodomain interface

Intrinsically disordered regions regulate RhlE RNA helicase functions in bacteria

HDAC4 influences the DNA damage response and counteracts senescence by assembling with HDAC1/HDAC2 to control H2BK120 acetylation and homology-directed repair

The impact of mRNA poly(A) tail length on eukaryotic translation stages

Correction to ‘hnRNPA1 couples nuclear export and translation of specific mRNAs downstream of FGF-2/S6K2 signalling’
Staphylococcal aconitase expression during iron deficiency is controlled by an srna-driven feedforward loop and moonlighting activity.

YY1 is involved in homologous recombination inhibition at guanine quadruplex sites in human cells

FRET-guided modeling of nucleic acids

Apollon: a deoxyribozyme that generates a yellow product

Engineering spacer specificity of the Cre/loxP system

Eukaryotic AlaX provides multiple checkpoints for quality and quantity of aminoacyl-tRNAs in translation

Concerted action of ataxin-2 and PABPC1-bound mRNA poly(A) tail in the formation of stress granules

Loops are geometric catalysts for DNA integration

Transfer learning for cross-context prediction of protein expression from 5’UTR sequence

Bacterial histone HBb from Bdellovibrio bacteriovorus compacts DNA by bending

Mapping the structural landscape of the yeast Ty3 retrotransposon RNA genome

OptoLacI: optogenetically engineered lactose operon repressor LacI responsive to light instead of IPTG

Aurora: a fluorescent deoxyribozyme for high-throughput screening

Crystal structure of a tetrameric RNA G-quadruplex formed by hexanucleotide repeat expansions of C9orf72 in ALS/FTD

Correction to ‘YASS: enhancing the sensitivity of DNA similarity search’
Induro-rt mediated circrna-sequencing (imcr-seq) enables comprehensive profiling of full-length and long circular rnas from low input total rna.

Principles of miRNA/miRNA* function in plant MIRNA processing

CAbiNet: joint clustering and visualization of cells and genes for single-cell transcriptomics

The relationship between nanoscale genome organization and gene expression in mouse embryonic stem cells during pluripotency transition

KDM6A–SND1 interaction maintains genomic stability by protecting the nascent DNA and contributes to cancer chemoresistance

Variable patterns of retrotransposition in different HeLa strains provide mechanistic insights into SINE RNA mobilization processes

iSuRe-HadCre is an essential tool for effective conditional genetics

AlphaKnot 2.0: a web server for the visualization of proteins’ knotting and a database of knotted AlphaFold-predicted models

Adaptation of a eukaryote-like ProRS to a prokaryote-like tRNA Pro

The 5′-terminal stem–loop RNA element of SARS-CoV-2 features highly dynamic structural elements that are sensitive to differences in cellular pH

Structural characterization of two prototypical repressors of SorC family reveals tetrameric assemblies on DNA and mechanism of function

Insights into the molecular mechanism of ParAB S system in chromosome partition by Hp ParA and Hp ParB

Cooperation of regulatory RNA and the RNA degradosome in transcript surveillance

YjgA plays dual roles in enhancing PTC maturation

Replication protein A dynamically re-organizes on primer/template junctions to permit DNA polymerase δ holoenzyme assembly and initiation of DNA synthesis

Liam tackles complex multimodal single-cell data integration challenges

Increased transcriptional elongation and RNA stability of GPCR ligand binding genes unveiled via RNA polymerase II degradation

Correction to ‘ChemFH: an integrated tool for screening frequent false positives in chemical biology and drug discovery’
Chemfree: a one-stop comprehensive platform for ecological and environmental risk evaluation of chemicals in one health world.

Structure of the E. coli nucleoid-associated protein YejK reveals a novel DNA binding clamp

Concurrent D-loop cleavage by Mus81 and Yen1 yields half-crossover precursors

In silico design of DNA sequences for in vivo nucleosome positioning

Modular CRISPR/Cas12a synergistic activation platform for detection and logic operations

Thank you for visiting nature.com. You are using a browser version with limited support for CSS. To obtain the best experience, we recommend you use a more up to date browser (or turn off compatibility mode in Internet Explorer). In the meantime, to ensure continued support, we are displaying the site without styles and JavaScript.
- View all journals
- Explore content
- About the journal
- Publish with us
- Sign up for alerts
Collection
Nucleic acid chemistry
Since the discovery of the double-helical structure of DNA and postulation of the central dogma of molecular biology, stating that the flow of genetic information goes from DNA to RNA to protein, the field of nucleic acid chemistry has expanded dramatically.
Nucleic acids have become important diagnostic markers for many diseases, enabled by breakthroughs in synthesis and sequencing technologies.
Nucleic acids have become medical modalities. We have recently witnessed the admission of antisense oligonucleotides to cure genetic diseases and the rapid development of mRNA vaccines against Covid-19 during the pandemic in 2020.
These developments are enabled by nucleic acid chemistry, the ability to synthesize oligonucleotides and install modifications at will. The field benefits from a tight interconnection between purely synthetic chemistry and molecular biology, or a combination of both as in chemo-enzymatic methods.
More and more functions of nucleic acids are discovered in vitro and in cells, such as ribozymes selected to catalyze methylation reactions and ribozymes cutting DNA in genomes.
DNA was shown to contain not only the four canonical nucleobases A, C, G, T but also methylated versions of C and their oxidized forms. The repertoire of RNA modifications is still expanding, with >170 currently annotated ones. The analysis, quantification, and mapping of these modifications on a transcriptome-wide scale is a prerequisite to understand their function and relevance in health and disease. Their exact function and dynamic aspects are only starting to be understood.
The already diverse natural functions of nucleic acids, behaving as aptamers, riboswitches, ribozymes, and DNAzymes can be further expanded by various natural and non-natural functionalities, such as tags, probes, markers, or drug molecules that can be installed in DNA or RNA. Such functionalized nucleic acids can be exploited for broad applications in gene editing, synthetic biology, biosensing, and drug discovery.
This Collection aims to offer insights and inspiration in the field of nucleic acid chemistry, including but not limited to:
- Synthesis, modifications, functionalizations and bioconjugations of nucleic acids
- Detection, biochemical profiling and structural characterization of nucleic acids
- Application of nucleic acids in chemical biology, medicine, diagnostics and more.
We welcome both fundamental and applied studies, as well as both experimental and theoretical research.
The Collection primarily welcomes original research papers, and we encourage submissions from all authors—and not by invitation only.
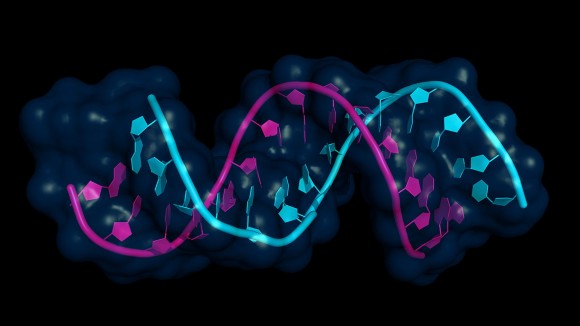
Andrea Rentmeister, PhD
Department of Chemistry, Ludwig-Maximilians-Universität München, Germany
Michal Hocek, PhD, DSc
Institute of Organic Chemistry and Biochemistry of the Czech Academy of Sciences, Czech Republic
- Collection content
- How to submit
- About the Guest Editors
- About this Collection
Synthesis and modification of nucleic acids
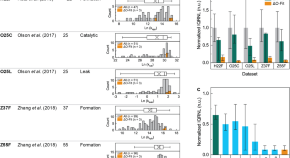
Generation of DNA oligomers with similar chemical kinetics via in-silico optimization
Networks of interacting DNA oligomers have various applications in molecular biology, chemistry and materials science, however, kinetic dispersions during DNA hybridization can be problematic for some applications. Here, the authors reveal that limiting unnecessary duplexes using in-silico optimization can reduce in-vitro kinetic dispersions by as much as 96%.
- Michael Tobiason
- Bernard Yurke
- William L. Hughes

The selection of a hydrophobic 7-phenylbutyl-7-deazaadenine-modified DNA aptamer with high binding affinity for the Heat Shock Protein 70
DNA aptamers can be selected against a wide range of therapeutic targets, however, the success rate of selective binding remains low due to the highly hydrophilic nature of the DNA backbone. Here, the authors design a hydrophobic 7-phenylbutyl-7-deazaadenine-modified DNA aptamer showing high binding affinity for the heat shock protein 70.
- Catherine Mulholland
- Ivana Jestřábová
- Michal Hocek
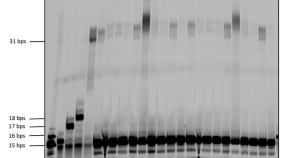
Evaluation of 3′-phosphate as a transient protecting group for controlled enzymatic synthesis of DNA and XNA oligonucleotides
Controlled enzymatic DNA synthesis represents an alternative synthetic methodology that circumvents the limitations of traditional soild-phase synthesis. Here, the authors explore the use of 3’-phosphate as a transient protecting group for the controlled enzymatic synthesis of DNA and XNA oligonucleotides.
- Marie Flamme
- Steven Hanlon
- Marcel Hollenstein
Structure and function of nucleic acids
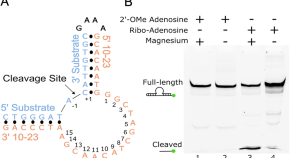
Structure of a 10-23 deoxyribozyme exhibiting a homodimer conformation
RNA-cleaving DNAzymes exhibit potential as biosensors and in vivo knockdown agents, however, the structures of DNAzymes remain underexplored. Here, the authors report the 2.7 Å X-Ray crystal structure of a 10-23 DNAzyme–substrate complex in a homodimer conformation.
- Evan R. Cramer
- Sarah A. Starcovic
- Aaron R. Robart
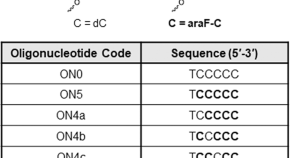
i-Motif folding intermediates with zero-nucleotide loops are trapped by 2′-fluoroarabinocytidine via F···H and O···H hydrogen bonds
The oligonucleotide d(TC 5 ) forms a well-characterized tetrameric i-motif in solution; however, the isolation of dimeric and trimeric intermediates remains challenging. Here, the authors report that 2′-deoxy-2′-fluoroarabinocytidine substitutions can prompt TC 5 to form dimeric i-motif folding intermediates through fluorine and oxygen hydrogen bonds.
- Roberto El-Khoury
- Veronica Macaluso
- Masad J. Damha
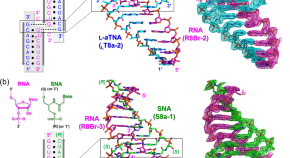
Intrastrand backbone-nucleobase interactions stabilize unwound right-handed helical structures of heteroduplexes of L- a TNA/RNA and SNA/RNA
Serinol nucleic acid and L-threoninol nucleic acid can bind to RNA and DNA, endowing them with potential as nucleic acid-based drugs. Here the authors prepare single crystals of L- a TNA/RNA and SNA/RNA heteroduplexes to further our structural understanding of how synthetic nucleic acids hybridize with natural nucleic acids.
- Yukiko Kamiya
- Tadashi Satoh
- Hiroyuki Asanuma
Applications of nucleic acids in chemical biology and medicinal chemistry
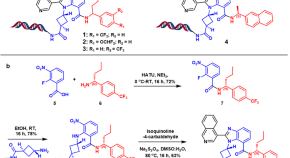
DNA-encoded chemical libraries yield non-covalent and non-peptidic SARS-CoV-2 main protease inhibitors
Conventional structure-based design of M pro inhibitors of SARS-CoV-2 often starts from the structural information of M pro and their binders; however, the continual rise of resistant strains requires innovative routes to discover new inhibitors. Here, the authors develop a DNA-encoded chemical library screening to produce non-covalent, non-peptidic small molecule inhibitors for SARS-CoV-2 M pro independently of preliminary knowledge regarding suitable starting points.
- Ravikumar Jimmidi
- Srinivas Chamakuri
- Damian W. Young
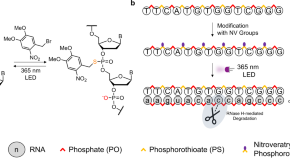
Accessible light-controlled knockdown of cell-free protein synthesis using phosphorothioate-caged antisense oligonucleotides
Light-activated antisense oligonucleotides have been developed to induce gene knockdown in living cells, however, their synthesis remains challenging and application in cell-free systems is underexplored. Here, the authors report a one-step method for selectively attaching photocages onto phosphorothioate linkages of antisense oligonucleotides that can knockdown cell-free protein synthesis using light.
- Denis Hartmann
- Michael J. Booth
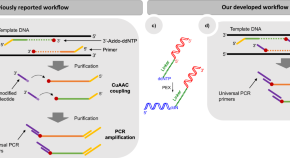
Advanced preparation of fragment libraries enabled by oligonucleotide-modified 2′,3′-dideoxynucleotides
Next-generation genome sequencing technologies have revolutionized the life sciences, however all sequencing platforms require nucleic acid pre-processing to generate suitable libraries for sequencing. Here, oligonucleotide-tethered 2′,3′-dideoxynucleotide terminators bearing universal priming sites are synthesised and incorporated by DNA polymerases, allowing integration of the fragmentation step into the library preparation workflow while also enabling the obtained fragments to be readily labeled by platform-specific adapters.
- Justina Medžiūnė
- Žana Kapustina
- Arvydas Lubys
Quick links
- Explore articles by subject
- Guide to authors
- Editorial policies

This website uses cookies to ensure you get the best experience. Learn more about DOAJ’s privacy policy.
Hide this message
You are using an outdated browser. Please upgrade your browser to improve your experience and security.
The Directory of Open Access Journals
Quick search, nucleic acids research nar.
0305-1048 (Print) / 1362-4962 (Online)
- ISSN Portal
Publishing with this journal
The journal charges up to:
as publication fees (article processing charges or APCs).
There is a waiver policy for these charges.
Look up the journal's:
- Aims & scope
- Instructions for authors
- Editorial Board
- Anonymous peer review
→ This journal checks for plagiarism .
Expect on average 7 weeks from submission to publication.
Best practice
This journal began publishing in open access in 2005 . What does DOAJ define as Open Accesss?
This journal uses a CC BY or a CC BY-NC license.
Attribution Attribution Non-Commercial
→ Look up their open access statement and their license terms .
The author does not retain unrestricted copyrights and publishing rights.
→ Learn more about their copyright policy .
Articles digitally archived in:
- Scholars Portal
→ Find out about their archiving policy .
Deposit policy with:
- Sherpa/Romeo
Permanent article identifier:
Journal metadata
Publisher Oxford University Press , United Kingdom Manuscripts accepted in English
LCC subjects Look up the Library of Congress Classification Outline Science: Biology (General): Genetics Keywords dna biochemistry computational biology genomics molecular biology rna
WeChat QR code
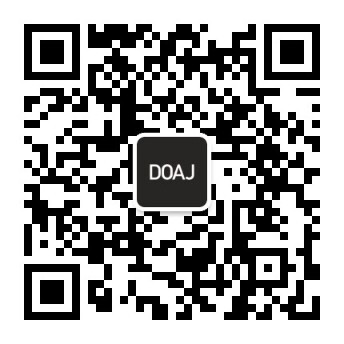
- Search Menu
- Sign in through your institution
- Chemical Biology and Nucleic Acid Chemistry
- Computational Biology
Critical Reviews and Perspectives
- Data Resources and Analyses
- Gene Regulation, Chromatin and Epigenetics
- Genome Integrity, Repair and Replication
- Methods Online
- Molecular Biology
- Nucleic Acid Enzymes
- RNA and RNA-protein complexes
- Structural Biology
- Synthetic Biology and Bioengineering
- Advance Articles
Breakthrough Articles
- Special Collections
- Scope and Criteria for Consideration
- Author Guidelines
- Data Deposition Policy
- Database Issue Guidelines
- Web Server Issue Guidelines
- Submission Site
- About Nucleic Acids Research
- Editors & Editorial Board
- Information of Referees
- Self-Archiving Policy
- Dispatch Dates
- Advertising and Corporate Services
- Journals Career Network
- Journals on Oxford Academic
- Books on Oxford Academic
Latest Articles

Senior Executive Editors of Nucleic Acids Research
Julian Sale MRC Laboratory of Molecular Biology, Cambridge, UK
Barry Stoddard Fred Hutchinson Cancer Research Center, Seattle, WA, USA
View full list of Editors & Editorial Board members

Celebrating 50 years of NAR
Nucleic Acids Research is celebrating its 50th anniversary this year. We owe our success to the incredible work of the three founding editors, Richard T. Walker and A. Stanley Jones at the University of Birmingham in the UK, and Dieter Söll at Yale University in the USA. Their vision and dedication have been carried forward by the editors who followed them, as well as our authors and reviewers. Today, NAR remains one of the leading journals in the biological sciences.
Celebrate our 50th anniversary with us
More from Nucleic Acids Research

CRISPR in Nucleic Acids Research : a sequel collection
In 2016, NAR assembled a special collection of studies, published in its pages over the preceding five-years, that described the biology and use of CRISPR/Cas systems. Now, eight years later and as part of NAR ’s 50th anniversary celebration, we have reviewed our subsequent content and identified almost 500 articles focused upon or utilizing CRISPR systems that have been published in NAR since that early collection. The titles that we have chosen to highlight represent some of the most impactful of these studies.
Browse the collection

NAR 's Breakthrough articles present high-impact studies answering long-standing questions in the field of nucleic acids research and/or open up new areas and mechanistic hypothesis for investigation.
Explore the best papers published with NAR

2024 Database issue
The 2024 Nucleic Acids Research Database issue contains 180 papers, including 90 papers reporting on new databases and 83 updates from resources previously published in the issue. Seven additional manuscripts provide updates on databases most recently published elsewhere. The issue also includes a Breakthrough Article reporting on the Novel Metagenome Protein Families Database (NMPFamsDB).
View the issue

2023 Web Server issue
The 2023 Nucleic Acids Research Web Server issue is the 21st in a series of annual issues dedicated to web-based software resources for analysis and visualization of molecular biology data. This issue includes 81 articles covering web servers that support research activities in a wide range of areas, ranging from software aimed at the wet lab through structural biology to computational methods.
Publishing with Nucleic Acids Research
Nucleic acids research : 50 years of research for scientists by scientists.
NAR 's 50 years of forming relationships with our author community has allowed the journal to continue paving the way with cutting-edge, reputable research in the nucleic acids and protein fields.
View our information for authors
NAR was the first OUP journal to flip to fully open access
NAR embraces and endorses the principles of Open Science, a movement that promotes the unhindered access to scientific research. The benefits of publishing open access include:
- Greater visibility and impact of research
- Increased citation and usage
- Compliance with funder mandates
- Increased engagement with a more public audience
Learn more about NAR 's open access initiative
Promote your published work with NAR
As the author, you are the best advocate for your work and we encourage you to be involved in promoting your publication! Sharing your ideas and news about your publication with your colleagues and friends could take as little as 15 minutes and will make a real difference in raising the profile of your research.
View OUP's self-promotion guide & learn how OUP also works to promote your content
Read and publish agreements
Oxford University Press has Read and Publish agreements with over 25 institutions and consortia around the world allowing affiliated researchers at participating institutions to:
- publish accepted articles open access in OUP journal, with their institutions covering the open access publication charge
- read high quality, high impact research from OUP's prestigious journals
Find out of your institution is participating
Article Types

Standard Research Articles
Standard research articles are published on a variety of subject categories including chemical biology and nucleic acid chemistry, computational biology, data resources and analyses, gene regulation, chromatin and epigenetics, molecular biology, RNA and RNA-protein complexes, structural biology and more.
- Browse this article type
- Learn more about publishing this article type

Methods Articles
Nucleic Acids Research publishes methods manuscripts that detail methodological developments of highest originality and usefulness within NAR 's core subject areas. Methods papers should report novel techniques, significant advances in existing techniques, and/or demonstration novel utility or advantage to an extended, rather than a specialist, audience.
- Learn more about publishing the article type

Critical Reviews and Perspectives are dedicated to reviews relevant to the journal's core areas of interest in DNA/RNA function and the structure and interactions of proteins involved in nucleic acid interactions. These papers are by invitation only, but NAR welcomes unsolicited proposals.

Database & Web Server Articles
Nucleic Acids Research publishes two special online issues, a biological databases one in January of each year, and a web-based software resources of value to the biological community one in July of each year.
Database articles
Web server articles.

Meet our NEW Early Career Investigator Advisory Board!
The Early Career Investigator (ECI) Advisory Board for Nucleic Acids Research represents an esteemed group of ECIs working in physical, chemical, or biological aspects of nucleic acids. Our board work closely with the journal's Executive Editor team to ensure that NAR remains a leading, community-focused, and scientist-led resource for our next generation of researchers in this field. ECIs participate in a dedicated editorial mentoring scheme for a two-year term.
Meet the appointed members

Nobel Prize winning research from NAR authors
Congratulations to Katalin Karikó and Drew Weissman on being awarded the 2023 Nobel Prize in Physiology or Medicine for their discoveries concerning nucleoside base modifications that enabled the development of effective mRNA vaccines against COVID-19.
Read the NAR article cited as a key publication in this research, ' Incorporation of pseudouridine into mRNA enhances translation by diminishing PKR activation '.

Email alerts
Register to receive email alerts as soon as new content from Nucleic Acids Research is published online.

NAR sister titles
In addition to Nucleic Acids Research , the following two journals are published under the NAR umbrella following the same commitment to open access, reputable, and cutting-edge research for scientists by scientists.
- NAR Genomics and Bioinformatics - focuses on genomics and bioinformatics large-scale data analysis
- NAR Cancer - focuses on research at the intersection of the nucleic acids research and cancer fields
- NAR Molecular Medicine - focuses on research at the intersection of nucleic acids research and molecular medicine
Related Titles
- Editorial Board
Affiliations
- Online ISSN 1362-4962
- Print ISSN 0305-1048
- Copyright © 2024 Oxford University Press
- About Oxford Academic
- Publish journals with us
- University press partners
- What we publish
- New features
- Open access
- Institutional account management
- Rights and permissions
- Get help with access
- Accessibility
- Advertising
- Media enquiries
- Oxford University Press
- Oxford Languages
- University of Oxford
Oxford University Press is a department of the University of Oxford. It furthers the University's objective of excellence in research, scholarship, and education by publishing worldwide
- Copyright © 2024 Oxford University Press
- Cookie settings
- Cookie policy
- Privacy policy
- Legal notice
This Feature Is Available To Subscribers Only
Sign In or Create an Account
This PDF is available to Subscribers Only
For full access to this pdf, sign in to an existing account, or purchase an annual subscription.
Nucleic Acids Research
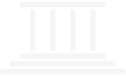
Subject Area and Category
Oxford University Press
Publication type
03051048, 13624962
Information
How to publish in this journal
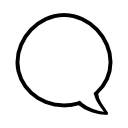
The set of journals have been ranked according to their SJR and divided into four equal groups, four quartiles. Q1 (green) comprises the quarter of the journals with the highest values, Q2 (yellow) the second highest values, Q3 (orange) the third highest values and Q4 (red) the lowest values.
Category | Year | Quartile |
---|---|---|
Genetics | 1999 | Q1 |
Genetics | 2000 | Q1 |
Genetics | 2001 | Q1 |
Genetics | 2002 | Q1 |
Genetics | 2003 | Q1 |
Genetics | 2004 | Q1 |
Genetics | 2005 | Q1 |
Genetics | 2006 | Q1 |
Genetics | 2007 | Q1 |
Genetics | 2008 | Q1 |
Genetics | 2009 | Q1 |
Genetics | 2010 | Q1 |
Genetics | 2011 | Q1 |
Genetics | 2012 | Q1 |
Genetics | 2013 | Q1 |
Genetics | 2014 | Q1 |
Genetics | 2015 | Q1 |
Genetics | 2016 | Q1 |
Genetics | 2017 | Q1 |
Genetics | 2018 | Q1 |
Genetics | 2019 | Q1 |
Genetics | 2020 | Q1 |
Genetics | 2021 | Q1 |
Genetics | 2022 | Q1 |
Genetics | 2023 | Q1 |
The SJR is a size-independent prestige indicator that ranks journals by their 'average prestige per article'. It is based on the idea that 'all citations are not created equal'. SJR is a measure of scientific influence of journals that accounts for both the number of citations received by a journal and the importance or prestige of the journals where such citations come from It measures the scientific influence of the average article in a journal, it expresses how central to the global scientific discussion an average article of the journal is.
Year | SJR |
---|---|
1999 | 4.690 |
2000 | 4.614 |
2001 | 3.852 |
2002 | 4.217 |
2003 | 4.395 |
2004 | 4.912 |
2005 | 5.092 |
2006 | 4.776 |
2007 | 5.100 |
2008 | 4.912 |
2009 | 5.669 |
2010 | 5.381 |
2011 | 5.976 |
2012 | 6.329 |
2013 | 6.801 |
2014 | 6.640 |
2015 | 7.358 |
2016 | 7.883 |
2017 | 9.025 |
2018 | 8.636 |
2019 | 8.907 |
2020 | 9.008 |
2021 | 8.241 |
2022 | 8.234 |
2023 | 7.048 |
Evolution of the number of published documents. All types of documents are considered, including citable and non citable documents.
Year | Documents |
---|---|
1999 | 729 |
2000 | 796 |
2001 | 701 |
2002 | 826 |
2003 | 1062 |
2004 | 1161 |
2005 | 1169 |
2006 | 1094 |
2007 | 1138 |
2008 | 1068 |
2009 | 1403 |
2010 | 759 |
2011 | 1218 |
2012 | 1414 |
2013 | 1308 |
2014 | 1521 |
2015 | 1374 |
2016 | 1160 |
2017 | 1421 |
2018 | 1272 |
2019 | 1262 |
2020 | 1109 |
2021 | 1494 |
2022 | 1270 |
2023 | 1171 |
This indicator counts the number of citations received by documents from a journal and divides them by the total number of documents published in that journal. The chart shows the evolution of the average number of times documents published in a journal in the past two, three and four years have been cited in the current year. The two years line is equivalent to journal impact factor ™ (Thomson Reuters) metric.
Cites per document | Year | Value |
---|---|---|
Cites / Doc. (4 years) | 1999 | 5.259 |
Cites / Doc. (4 years) | 2000 | 5.407 |
Cites / Doc. (4 years) | 2001 | 6.067 |
Cites / Doc. (4 years) | 2002 | 5.651 |
Cites / Doc. (4 years) | 2003 | 6.212 |
Cites / Doc. (4 years) | 2004 | 7.142 |
Cites / Doc. (4 years) | 2005 | 7.753 |
Cites / Doc. (4 years) | 2006 | 7.726 |
Cites / Doc. (4 years) | 2007 | 7.997 |
Cites / Doc. (4 years) | 2008 | 7.302 |
Cites / Doc. (4 years) | 2009 | 7.927 |
Cites / Doc. (4 years) | 2010 | 7.650 |
Cites / Doc. (4 years) | 2011 | 8.548 |
Cites / Doc. (4 years) | 2012 | 9.393 |
Cites / Doc. (4 years) | 2013 | 9.654 |
Cites / Doc. (4 years) | 2014 | 9.660 |
Cites / Doc. (4 years) | 2015 | 9.984 |
Cites / Doc. (4 years) | 2016 | 10.216 |
Cites / Doc. (4 years) | 2017 | 11.154 |
Cites / Doc. (4 years) | 2018 | 11.090 |
Cites / Doc. (4 years) | 2019 | 12.576 |
Cites / Doc. (4 years) | 2020 | 15.137 |
Cites / Doc. (4 years) | 2021 | 17.443 |
Cites / Doc. (4 years) | 2022 | 17.648 |
Cites / Doc. (4 years) | 2023 | 16.233 |
Cites / Doc. (3 years) | 1999 | 5.259 |
Cites / Doc. (3 years) | 2000 | 5.933 |
Cites / Doc. (3 years) | 2001 | 5.533 |
Cites / Doc. (3 years) | 2002 | 6.170 |
Cites / Doc. (3 years) | 2003 | 6.702 |
Cites / Doc. (3 years) | 2004 | 7.292 |
Cites / Doc. (3 years) | 2005 | 8.000 |
Cites / Doc. (3 years) | 2006 | 7.950 |
Cites / Doc. (3 years) | 2007 | 7.785 |
Cites / Doc. (3 years) | 2008 | 7.223 |
Cites / Doc. (3 years) | 2009 | 8.112 |
Cites / Doc. (3 years) | 2010 | 7.720 |
Cites / Doc. (3 years) | 2011 | 8.900 |
Cites / Doc. (3 years) | 2012 | 9.663 |
Cites / Doc. (3 years) | 2013 | 9.262 |
Cites / Doc. (3 years) | 2014 | 9.962 |
Cites / Doc. (3 years) | 2015 | 10.186 |
Cites / Doc. (3 years) | 2016 | 10.514 |
Cites / Doc. (3 years) | 2017 | 11.471 |
Cites / Doc. (3 years) | 2018 | 11.487 |
Cites / Doc. (3 years) | 2019 | 12.597 |
Cites / Doc. (3 years) | 2020 | 14.850 |
Cites / Doc. (3 years) | 2021 | 18.884 |
Cites / Doc. (3 years) | 2022 | 18.085 |
Cites / Doc. (3 years) | 2023 | 14.418 |
Cites / Doc. (2 years) | 1999 | 5.705 |
Cites / Doc. (2 years) | 2000 | 5.298 |
Cites / Doc. (2 years) | 2001 | 5.991 |
Cites / Doc. (2 years) | 2002 | 6.679 |
Cites / Doc. (2 years) | 2003 | 6.683 |
Cites / Doc. (2 years) | 2004 | 7.532 |
Cites / Doc. (2 years) | 2005 | 8.167 |
Cites / Doc. (2 years) | 2006 | 7.506 |
Cites / Doc. (2 years) | 2007 | 7.559 |
Cites / Doc. (2 years) | 2008 | 7.246 |
Cites / Doc. (2 years) | 2009 | 8.109 |
Cites / Doc. (2 years) | 2010 | 7.786 |
Cites / Doc. (2 years) | 2011 | 8.943 |
Cites / Doc. (2 years) | 2012 | 8.359 |
Cites / Doc. (2 years) | 2013 | 9.659 |
Cites / Doc. (2 years) | 2014 | 9.941 |
Cites / Doc. (2 years) | 2015 | 10.309 |
Cites / Doc. (2 years) | 2016 | 10.533 |
Cites / Doc. (2 years) | 2017 | 11.989 |
Cites / Doc. (2 years) | 2018 | 11.080 |
Cites / Doc. (2 years) | 2019 | 11.335 |
Cites / Doc. (2 years) | 2020 | 15.987 |
Cites / Doc. (2 years) | 2021 | 19.770 |
Cites / Doc. (2 years) | 2022 | 14.444 |
Cites / Doc. (2 years) | 2023 | 15.172 |
Evolution of the total number of citations and journal's self-citations received by a journal's published documents during the three previous years. Journal Self-citation is defined as the number of citation from a journal citing article to articles published by the same journal.
Cites | Year | Value |
---|---|---|
Self Cites | 1999 | 1079 |
Self Cites | 2000 | 1179 |
Self Cites | 2001 | 974 |
Self Cites | 2002 | 1010 |
Self Cites | 2003 | 1394 |
Self Cites | 2004 | 1741 |
Self Cites | 2005 | 2225 |
Self Cites | 2006 | 1769 |
Self Cites | 2007 | 2242 |
Self Cites | 2008 | 1707 |
Self Cites | 2009 | 2702 |
Self Cites | 2010 | 1192 |
Self Cites | 2011 | 2358 |
Self Cites | 2012 | 2629 |
Self Cites | 2013 | 2186 |
Self Cites | 2014 | 2992 |
Self Cites | 2015 | 2797 |
Self Cites | 2016 | 2601 |
Self Cites | 2017 | 2715 |
Self Cites | 2018 | 2296 |
Self Cites | 2019 | 2284 |
Self Cites | 2020 | 2194 |
Self Cites | 2021 | 2946 |
Self Cites | 2022 | 2631 |
Self Cites | 2023 | 2259 |
Total Cites | 1999 | 13132 |
Total Cites | 2000 | 14276 |
Total Cites | 2001 | 13346 |
Total Cites | 2002 | 13735 |
Total Cites | 2003 | 15569 |
Total Cites | 2004 | 18878 |
Total Cites | 2005 | 24391 |
Total Cites | 2006 | 26966 |
Total Cites | 2007 | 26657 |
Total Cites | 2008 | 24565 |
Total Cites | 2009 | 26770 |
Total Cites | 2010 | 27860 |
Total Cites | 2011 | 28747 |
Total Cites | 2012 | 32660 |
Total Cites | 2013 | 31406 |
Total Cites | 2014 | 39252 |
Total Cites | 2015 | 43219 |
Total Cites | 2016 | 44189 |
Total Cites | 2017 | 46513 |
Total Cites | 2018 | 45430 |
Total Cites | 2019 | 48535 |
Total Cites | 2020 | 58730 |
Total Cites | 2021 | 68793 |
Total Cites | 2022 | 69897 |
Total Cites | 2023 | 55841 |
Evolution of the number of total citation per document and external citation per document (i.e. journal self-citations removed) received by a journal's published documents during the three previous years. External citations are calculated by subtracting the number of self-citations from the total number of citations received by the journal’s documents.
Cites | Year | Value |
---|---|---|
External Cites per document | 1999 | 4.827 |
External Cites per document | 2000 | 5.443 |
External Cites per document | 2001 | 5.129 |
External Cites per document | 2002 | 5.717 |
External Cites per document | 2003 | 6.102 |
External Cites per document | 2004 | 6.619 |
External Cites per document | 2005 | 7.270 |
External Cites per document | 2006 | 7.428 |
External Cites per document | 2007 | 7.131 |
External Cites per document | 2008 | 6.721 |
External Cites per document | 2009 | 7.293 |
External Cites per document | 2010 | 7.389 |
External Cites per document | 2011 | 8.170 |
External Cites per document | 2012 | 8.885 |
External Cites per document | 2013 | 8.617 |
External Cites per document | 2014 | 9.203 |
External Cites per document | 2015 | 9.527 |
External Cites per document | 2016 | 9.895 |
External Cites per document | 2017 | 10.801 |
External Cites per document | 2018 | 10.906 |
External Cites per document | 2019 | 12.004 |
External Cites per document | 2020 | 14.295 |
External Cites per document | 2021 | 18.075 |
External Cites per document | 2022 | 17.404 |
External Cites per document | 2023 | 13.835 |
Cites per document | 1999 | 5.259 |
Cites per document | 2000 | 5.933 |
Cites per document | 2001 | 5.533 |
Cites per document | 2002 | 6.170 |
Cites per document | 2003 | 6.702 |
Cites per document | 2004 | 7.292 |
Cites per document | 2005 | 8.000 |
Cites per document | 2006 | 7.950 |
Cites per document | 2007 | 7.785 |
Cites per document | 2008 | 7.223 |
Cites per document | 2009 | 8.112 |
Cites per document | 2010 | 7.720 |
Cites per document | 2011 | 8.900 |
Cites per document | 2012 | 9.663 |
Cites per document | 2013 | 9.262 |
Cites per document | 2014 | 9.962 |
Cites per document | 2015 | 10.186 |
Cites per document | 2016 | 10.514 |
Cites per document | 2017 | 11.471 |
Cites per document | 2018 | 11.487 |
Cites per document | 2019 | 12.597 |
Cites per document | 2020 | 14.850 |
Cites per document | 2021 | 18.884 |
Cites per document | 2022 | 18.085 |
Cites per document | 2023 | 14.418 |
International Collaboration accounts for the articles that have been produced by researchers from several countries. The chart shows the ratio of a journal's documents signed by researchers from more than one country; that is including more than one country address.
Year | International Collaboration |
---|---|
1999 | 22.63 |
2000 | 23.24 |
2001 | 27.96 |
2002 | 20.82 |
2003 | 23.26 |
2004 | 23.00 |
2005 | 25.06 |
2006 | 21.02 |
2007 | 26.19 |
2008 | 27.06 |
2009 | 30.51 |
2010 | 30.30 |
2011 | 36.54 |
2012 | 33.45 |
2013 | 32.80 |
2014 | 37.08 |
2015 | 38.28 |
2016 | 39.57 |
2017 | 39.76 |
2018 | 41.59 |
2019 | 41.05 |
2020 | 38.23 |
2021 | 36.88 |
2022 | 35.59 |
2023 | 35.10 |
Not every article in a journal is considered primary research and therefore "citable", this chart shows the ratio of a journal's articles including substantial research (research articles, conference papers and reviews) in three year windows vs. those documents other than research articles, reviews and conference papers.
Documents | Year | Value |
---|---|---|
Non-citable documents | 1999 | 14 |
Non-citable documents | 2000 | 15 |
Non-citable documents | 2001 | 13 |
Non-citable documents | 2002 | 4 |
Non-citable documents | 2003 | 6 |
Non-citable documents | 2004 | 9 |
Non-citable documents | 2005 | 10 |
Non-citable documents | 2006 | 11 |
Non-citable documents | 2007 | 11 |
Non-citable documents | 2008 | 12 |
Non-citable documents | 2009 | 9 |
Non-citable documents | 2010 | 8 |
Non-citable documents | 2011 | 6 |
Non-citable documents | 2012 | 6 |
Non-citable documents | 2013 | 6 |
Non-citable documents | 2014 | 4 |
Non-citable documents | 2015 | 7 |
Non-citable documents | 2016 | 6 |
Non-citable documents | 2017 | 9 |
Non-citable documents | 2018 | 8 |
Non-citable documents | 2019 | 8 |
Non-citable documents | 2020 | 7 |
Non-citable documents | 2021 | 6 |
Non-citable documents | 2022 | 6 |
Non-citable documents | 2023 | 10 |
Citable documents | 1999 | 2483 |
Citable documents | 2000 | 2391 |
Citable documents | 2001 | 2399 |
Citable documents | 2002 | 2222 |
Citable documents | 2003 | 2317 |
Citable documents | 2004 | 2580 |
Citable documents | 2005 | 3039 |
Citable documents | 2006 | 3381 |
Citable documents | 2007 | 3413 |
Citable documents | 2008 | 3389 |
Citable documents | 2009 | 3291 |
Citable documents | 2010 | 3601 |
Citable documents | 2011 | 3224 |
Citable documents | 2012 | 3374 |
Citable documents | 2013 | 3385 |
Citable documents | 2014 | 3936 |
Citable documents | 2015 | 4236 |
Citable documents | 2016 | 4197 |
Citable documents | 2017 | 4046 |
Citable documents | 2018 | 3947 |
Citable documents | 2019 | 3845 |
Citable documents | 2020 | 3948 |
Citable documents | 2021 | 3637 |
Citable documents | 2022 | 3859 |
Citable documents | 2023 | 3863 |
Ratio of a journal's items, grouped in three years windows, that have been cited at least once vs. those not cited during the following year.
Documents | Year | Value |
---|---|---|
Uncited documents | 1999 | 347 |
Uncited documents | 2000 | 368 |
Uncited documents | 2001 | 331 |
Uncited documents | 2002 | 283 |
Uncited documents | 2003 | 287 |
Uncited documents | 2004 | 269 |
Uncited documents | 2005 | 294 |
Uncited documents | 2006 | 303 |
Uncited documents | 2007 | 294 |
Uncited documents | 2008 | 282 |
Uncited documents | 2009 | 259 |
Uncited documents | 2010 | 334 |
Uncited documents | 2011 | 267 |
Uncited documents | 2012 | 214 |
Uncited documents | 2013 | 232 |
Uncited documents | 2014 | 254 |
Uncited documents | 2015 | 280 |
Uncited documents | 2016 | 269 |
Uncited documents | 2017 | 270 |
Uncited documents | 2018 | 324 |
Uncited documents | 2019 | 320 |
Uncited documents | 2020 | 306 |
Uncited documents | 2021 | 229 |
Uncited documents | 2022 | 289 |
Uncited documents | 2023 | 372 |
Cited documents | 1999 | 2150 |
Cited documents | 2000 | 2038 |
Cited documents | 2001 | 2081 |
Cited documents | 2002 | 1943 |
Cited documents | 2003 | 2036 |
Cited documents | 2004 | 2320 |
Cited documents | 2005 | 2755 |
Cited documents | 2006 | 3089 |
Cited documents | 2007 | 3130 |
Cited documents | 2008 | 3119 |
Cited documents | 2009 | 3041 |
Cited documents | 2010 | 3275 |
Cited documents | 2011 | 2963 |
Cited documents | 2012 | 3166 |
Cited documents | 2013 | 3159 |
Cited documents | 2014 | 3686 |
Cited documents | 2015 | 3963 |
Cited documents | 2016 | 3934 |
Cited documents | 2017 | 3785 |
Cited documents | 2018 | 3631 |
Cited documents | 2019 | 3533 |
Cited documents | 2020 | 3649 |
Cited documents | 2021 | 3414 |
Cited documents | 2022 | 3576 |
Cited documents | 2023 | 3501 |
Evolution of the percentage of female authors.
Year | Female Percent |
---|---|
1999 | 29.11 |
2000 | 28.38 |
2001 | 28.97 |
2002 | 29.91 |
2003 | 31.13 |
2004 | 29.38 |
2005 | 30.03 |
2006 | 30.44 |
2007 | 31.03 |
2008 | 31.11 |
2009 | 32.78 |
2010 | 32.90 |
2011 | 32.52 |
2012 | 33.88 |
2013 | 33.98 |
2014 | 34.14 |
2015 | 35.07 |
2016 | 34.57 |
2017 | 36.47 |
2018 | 36.00 |
2019 | 37.47 |
2020 | 37.54 |
2021 | 38.16 |
2022 | 38.47 |
2023 | 39.29 |
Evolution of the number of documents cited by public policy documents according to Overton database.
Documents | Year | Value |
---|---|---|
Overton | 1999 | 28 |
Overton | 2000 | 29 |
Overton | 2001 | 30 |
Overton | 2002 | 50 |
Overton | 2003 | 56 |
Overton | 2004 | 50 |
Overton | 2005 | 61 |
Overton | 2006 | 54 |
Overton | 2007 | 61 |
Overton | 2008 | 56 |
Overton | 2009 | 81 |
Overton | 2010 | 32 |
Overton | 2011 | 66 |
Overton | 2012 | 84 |
Overton | 2013 | 67 |
Overton | 2014 | 90 |
Overton | 2015 | 68 |
Overton | 2016 | 54 |
Overton | 2017 | 47 |
Overton | 2018 | 33 |
Overton | 2019 | 39 |
Overton | 2020 | 34 |
Overton | 2021 | 22 |
Overton | 2022 | 8 |
Overton | 2023 | 1 |
Evoution of the number of documents related to Sustainable Development Goals defined by United Nations. Available from 2018 onwards.
Documents | Year | Value |
---|---|---|
SDG | 2018 | 226 |
SDG | 2019 | 222 |
SDG | 2020 | 207 |
SDG | 2021 | 288 |
SDG | 2022 | 238 |
SDG | 2023 | 240 |
Leave a comment
Name * Required
Email (will not be published) * Required
* Required Cancel
The users of Scimago Journal & Country Rank have the possibility to dialogue through comments linked to a specific journal. The purpose is to have a forum in which general doubts about the processes of publication in the journal, experiences and other issues derived from the publication of papers are resolved. For topics on particular articles, maintain the dialogue through the usual channels with your editor.
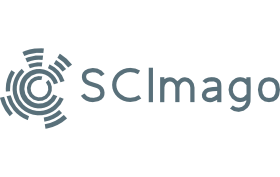
Follow us on @ScimagoJR Scimago Lab , Copyright 2007-2024. Data Source: Scopus®

Cookie settings
Cookie Policy
Legal Notice
Privacy Policy

An official website of the United States government
The .gov means it’s official. Federal government websites often end in .gov or .mil. Before sharing sensitive information, make sure you’re on a federal government site.
The site is secure. The https:// ensures that you are connecting to the official website and that any information you provide is encrypted and transmitted securely.
- Publications
- Account settings
Preview improvements coming to the PMC website in October 2024. Learn More or Try it out now .
- Advanced Search
- Journal List
- Portland Press Opt2Pay

Understanding biochemistry: structure and function of nucleic acids
Steve minchin.
School of Biosciences, University of Birmingham, Birmingham, United Kingdom
Julia Lodge
Nucleic acids, deoxyribonucleic acid (DNA) and ribonucleic acid (RNA), carry genetic information which is read in cells to make the RNA and proteins by which living things function. The well-known structure of the DNA double helix allows this information to be copied and passed on to the next generation. In this article we summarise the structure and function of nucleic acids. The article includes a historical perspective and summarises some of the early work which led to our understanding of this important molecule and how it functions; many of these pioneering scientists were awarded Nobel Prizes for their work. We explain the structure of the DNA molecule, how it is packaged into chromosomes and how it is replicated prior to cell division. We look at how the concept of the gene has developed since the term was first coined and how DNA is copied into RNA (transcription) and translated into protein (translation).
The structure of deoxyribonucleic acid
Deoxyribonucleic acid (DNA) is one of the most important molecules in living cells. It encodes the instruction manual for life. Genome is the complete set of DNA molecules within the organism, so in humans this would be the DNA present in the 23 pairs of chromosomes in the nucleus plus the relatively small mitochondrial genome. Humans have a diploid genome, inheriting one set of chromosomes from each parent. A complete and functioning diploid genome is required for normal development and to maintain life.
Discovery and chemical characterisation of DNA
DNA was discovered in 1869 by a Swiss biochemist, Friedrich Miescher. He wanted to determine the chemical composition of leucocytes (white blood cells), his source of leucocytes was pus from fresh surgical bandages. Although initially interested in all the components of the cell, Miescher quickly focussed on the nucleus because he observed that when treated with acid, a precipitate was formed which he called ‘nuclein’. Almost all molecular bioscience graduates would have repeated a form of this experiment in laboratory classes where DNA is isolated from cells. Miescher, Richard Altmann and Albrecht Kossel further characterised ‘nuclein’ and the name was changed to nucleic acid by Altmann. Kossel went on to show that nucleic acid contained purine and pyrimidine bases, a sugar and phosphate. Work in the 1930s from many scientists further characterised nucleic acids including the identification of the four bases and the presence of deoxyribose, hence the name deoxyribonucleic acid (DNA). Erwin Chargaff had found that DNA molecules from a particular species always contained the same amount of the bases cytosine (C) and guanine (G) and the same amount of adenosine (A) and thymine (T). So, for example, the human genome contains 20% C, 20% G, 30% A and 30% T.
DNA is a polymer made of monomeric units called nucleotides ( Figure 1 A), a nucleotide comprises a 5-carbon sugar, deoxyribose, a nitrogenous base and one or more phosphate groups. The building blocks for DNA synthesis contain three phosphate groups, two are lost during this process, so the DNA strand contains one phosphate group per nucleotide.

( A ) A nucleotide (guanosine triphosphate). The nitrogenous base (guanine in this example) is linked to the 1′ carbon of the deoxyribose and the phosphate groups are linked to the 5′ carbon. A nucleoside is a base linked to a sugar. A nucleotide is a nucleoside with one or more phosphate groups. ( B ) A DNA strand containing four nucleotides with the nitrogenous bases thymine (T), cytosine (C), adenine (A) and guanine (G) respectively. The 3′ carbon of one nucleotide is linked to the 5′ carbon of the next via a phosphodiester bond. The 5′ end is at the top and the 3′ end at the bottom.
There are four different bases in DNA, the double-ring purine bases: adenine and guanine; and the single-ring pyrimidine bases: cytosine and thymine ( Figure 1 B). The carbon within the deoxyribose ring are numbered 1′ to 5′. Within each monomer the phosphate is linked to the 5′ carbon of deoxyribose and the nitrogenous base is linked to the 1′ carbon, this is called an N-glyosidic bond. The phosphate group is acidic, hence the name nucleic acid.
In the DNA chain ( Figure 1 B), the phosphate residue forms a link between the 3′-hydroxyl of one deoxyribose and the 5′-hydroxyl of the next. This linkage is called a phosphodiester bond. DNA strands have a ‘sense of direction’. The deoxyribose at the top of the diagram in Figure 1 B is not linked to another deoxyribose; it terminates with a 5′ phosphate group. At the other end the chain terminates with a 3′ hydroxyl.
DNA is the genetic material
Although many scientists, including Miescher, had observed that prior to cell division the amount of nucleic acid increased, it was not believed to be the genetic material until the work of Fredrick Griffith, Oswald Avery, Colin MacLeod and Maclyn McCarty. In 1928, Griffith showed that living cells could be transformed by extracts from heat-killed cells and that this transformation had the potential to permanently change the genetic makeup of the recipient cell. Griffith was working with two strains of the bacterium Streptococcus pneumoniae. The encapsulated so-called S strain is virulent, whereas the non-capsulated R strain is nonvirulent. If the S strain is injected subcutaneously into mice, the mice die, whereas, if either live R strain is injected or heat-killed S strain is injected, the mouse lives. However, if a mixture of live R strain and heat-killed S strain is injected into a mouse, the mouse will die, and live S strain can be isolated from the blood. So, in the Griffith experiment a component of the heat-killed S strain is transforming the R strain. In 1944, Avery, MacLeod and McCarty went on to show that it was DNA that could transform the avirulent bacterium. They isolated a crude DNA extract from the S strain and destroyed any protein, lipid, carbohydrate and ribonucleic acid (RNA) component and showed that this purified DNA could still transform the R strain. However, when the purified DNA was treated with DNAse, an enzyme that degrades DNA, transformation was lost.
Alfred Hershey and Martha Chase confirmed that DNA was the genetic material. They used a virus that infects bacteria called a bacteriophage. The bacteriophage contains a protein capsid surrounding a DNA molecule. They showed that when bacteriophage T2 infects Escherichia coli , it is the phage DNA, not protein, that enters the bacterial cell.
Determining the structure of DNA
Once it had been shown that DNA was the genetic material, there was a race to determine the three-dimensional structure of the DNA molecule. At King’s College London, Rosalind Franklin and Maurice Wilkins, having obtained data using X-ray diffraction, had proposed that DNA had a helical structure and Franklin had obtained a particularly good X-ray diffraction pattern. In Cambridge, James Watson and Francis Crick used model building together with data from a variety of sources including Franklin’s X-ray diffraction pattern and Chargaff’s base composition data to work out the now well-known double helix structure of DNA. Their work was published in Nature in 1953. The Watson–Crick structure is shown in Figure 2 A.

( A ) The DNA double helix, with the sugar phosphate backbone on the outside and the nitrogenous bases in the middle. ( B ) An A:T and a G:C base pair with the C1′ of the deoxyribose indicated by the arrow. Note that the C1′ of the deoxyribose is in the same position in all base pairs. In this figure, the atoms on the upper edge of the base pair face into the major groove and those facing lower edge face into the minor groove. The hydrogen bonds between the base pairs are indicated by the dotted line.
DNA is a two-stranded helical structure, the two strands run in opposite directions. In Figure 2 A, one strand is running 5′ to 3′ top to bottom, whereas the other strand is running 3′ to 5′ top to bottom. The helix is right-handed which means that if you are looking down the axis, the helix turns clockwise as it gets further away from you. The two chains interact via hydrogen bonds between pairs of bases with adenine always pairing with thymine, and guanine always pairing with cytosine. The Watson–Crick structure therefore accounts for and explains the Chargaff data which showed that there was always an equal amount of C and G and of A and T. The regular nature of the double helix comes about because the distance between the 1′ carbon of the deoxyribose on one strand and 1′ carbon of the opposite deoxyribose is always the same irrespective of the base pair ( Figure 2 B). The 1′ carbons of the deoxyribose opposing nucleotides do not lie directly opposite each other on the helical axis, this means that the two sugar–phosphate backbones are not equally spaced along the helical axis resulting in major and minor grooves.
The diameter of the helix is 2 nm, adjacent bases are separated by 0.34 nm (0.34 × 10 −9 m) and related by a rotation of 36°, this results in the helical structure repeating every 10 residues. DNA molecules are normally very long and the sequence of bases along the DNA chain is not restricted. For example, the genome of the bacterium E. coli is a single circular chromosome which contains 4.6 million base pairs (4.6 × 10 6 bp), this is therefore 1.6 mm long (4.6 × 10 6 × 0.34 × 10 −9 m). The human genome is made up of 24 distinct chromosomes, chromosomes 1–22 and the X and Y chromosomes present in the nucleus plus mitochondrial DNA. The nuclear chromosomes vary in size from approximately 50–250 × 10 6 bp, the mitochondrial DNA is 17 × 10 3 bp. The total length of a haploid human genome is 3 × 10 9 bp. Within a single human diploid cell, which contains 23 chromosome pairs there is 2 m of DNA. Based on the assumption that humans contain 3 trillion cells with a nucleus, if all the DNA from a single human individual was put end to end, it would reach to the sun and back approximately 20 times.
Another important class of nucleic acids is RNA, the roles of RNA molecules in the cell will be discussed below. Chemically RNA is similar to DNA, it is a chain of similar monomers. The building blocks are nucleotides containing the 5-carbon sugar ribose, a phosphate and a nitrogenous base. The phosphate is attached to the 5′ carbon of the ribose and the nitrogenous base to the 1′ carbon ( Figure 3 ). RNA contains four bases adenine, guanine, cytosine and uracil. RNA is more labile (easily broken down) than DNA and most RNA molecules do not form stable secondary structures, some notable exceptions will be discussed below. The properties of RNA make it ideal as a genetic messenger during protein synthesis, the idea of this genetic messenger, mRNA, was proposed by François Jacob and Jacques Monod.

An RNA strand containing the four nucleotides with the nitrogenous bases: adenine (A), cytosine (C), guanine (G) and uracil (U) respectively. The 3′ carbon of the ribose of one nucleotide is linked to the 5′ carbon of the next via a phosphodiester bond. The 5′ end on the left and the 3′ end on the right.
Packaging of DNA into eukaryotic cells
DNA has to be highly condensed to fit into the bacterial cell or eukaryotic nucleus. In eukaryotes, histone proteins are used to condense the DNA into chromatin. The basic structure of chromatin is the nucleosome, a nucleosome contains DNA wrapped almost two times around the histone octamer (comprising two copies each of the histone proteins H2A, H2B, H3 and H4) ( Figure 4 ). Further levels of compaction are required to fit the DNA into the nucleus ( Figure 4 ), the nucleosomes are folded upon themselves to form the 30-nm fibre, this is then folded again to form the 300-nm fibre and during mitosis further compaction can occur forming the chromatid which is 700 nm in diameter.

Histone proteins (H2A, H2B, H3 and H4) associate to form a histone octamer. Approximately 147 bp of DNA wraps around histone octamer to form a nucleosome, generating a ‘beads on a string’ structure, the nucleosome together with histone H1 condense into the 30-nm fibre, there is further condensation to form the 300-nm fibre. During mitosis there is further compaction (not shown).
Processes such as DNA replication and DNA transcription need to occur in the chromatin environment and because of the level of compaction, this acts as a barrier to proteins that need to interact with DNA. Therefore, chromatin structure plays an important role in processes such as regulation of gene expression in eukaryotes. DNA and the histone proteins can be chemically modified, these are called epigenetic modifications as they do not change the DNA sequence, however, they can be passed on during cell division and to subsequent generations, a process known as epigenetic inheritance. As these epigenetic modifications can alter the chromatin structure they regulate gene transcription and can affect the phenotype. Epigenetics plays key roles in many processes, including development, cancer and behaviour and addiction. This will be discussed further later in this article.
Nuclear organisation plays an important role in many biological processes including regulation of gene transcription. In recent years the development of several techniques, including microscopy, have allowed us to gain an understanding of the way the genome is organised in 3D. Individual chromosomes are not randomly spaced within the nucleus; each chromosome has a distinct territory. Actively transcribed regions from different chromosomes are often close to each other and near the interior of the nucleus, whereas, inactive genes are on the periphery or near a special area called the nucleolus where ribosomal RNA is transcribed.
DNA replication
Whenever a cell divides there is a need to synthesise two copies of each chromosome present within the cell. For example in a human, prior to cell division, all 23 pairs of chromosomes need to be replicated to form 46 pairs, so that following cell division each daughter cell has a full complement (23 pairs) of chromosomes. The structure of DNA gives us a clue to how it is replicated, this was eloquently postulated by Watson and Crick in their 1953 paper: “It has not escaped our notice that the specific pairing we have postulated immediately suggests a possible copying mechanism for the genetic material”. Each strand can act as a template for the synthesis of the complementary strand, so the replication machinery would ‘unzip’ the double helix and read along the two existing ‘parent’ strands, synthesising a complementary new ‘daughter’ strand with A opposite T, C opposite G etc. This is described as semi-conservative, since each ‘new’ double-stranded DNA molecule has one original parent strand and one newly made daughter ‘strand’.
The evidence that DNA replication was semi-conservative came from an elegant experiment completed by Matthew Meselson and Franklin Stahl. They labelled the parental DNA with a heavy isotope of nitrogen ( 15 N) by growing bacteria in a growth medium that contained 15 NH 4 Cl. They then grew the bacteria, in a medium that contained 14 NH 4 Cl, in conditions such that any newly synthesised DNA would contain 14 N. Since DNA replication is semi-conservative, after one round of DNA replication, each cell would have a DNA molecule that contains one ‘old’ parental strand labelled with 15 N and one ‘new’ daughter strand labelled with 14 N. This was shown by analysing the density of the DNA using density-gradient centrifugation. As predicted, they observed that the new daughter DNA molecule had a density consistent with the fact that it contained both 15 N and 14 N and that this daughter DNA contained one strand with 15 N and another strand with 14 N.
DNA polymerase and DNA synthesis
The enzyme, DNA polymerase, is responsible for DNA synthesis. DNA polymerase is a template-driven enzyme, so it will use the parental DNA strand as a template. It cannot synthesise DNA in the absence of a template. In addition, it will only add nucleotides on to the 3′ end of an existing nucleic acid chain. The building blocks for DNA synthesis are deoxynucleoside triphosphates (dATP, dTTP, dCTP and dGTP). During DNA synthesis, the base within the incoming deoxynucleoside triphosphate pairs with the complementary base on the template strand, a phosphodiester bond is formed between the 5′ phosphate on the incoming nucleotide and the free 3′ hydroxyl on the existing nucleic acid chain; pyrophosphate is released ( Figure 5 ).

( A ) DNA polymerase binds the template DNA and the new strand. The next nucleotide to be added to the 3′ end of the growing chain will contain guanine (G), this is complementary to the C on the template strand. DNA polymerase catalyses the formation of a phosphodiester bond. ( B ) The chemical reaction during the formation of a phosphodiester bond, showing the addition of a nucleotide containing guanine and the release of pyrophosphate.
Pyrophosphate is the two phosphate residues within the deoxynucleoside triphosphate building block that are not incorporated into the DNA chain. DNA polymerase synthesises DNA in the 5′ to 3′ direction, because it can only add nucleotides on to the 3′ end of the chain. DNA polymerase has proofreading activity, so after the phosphodiester bond has been formed, the base pairing is checked and if a nucleotide with an incorrect base has been added, DNA polymerase will remove the nucleotide using a 3′ to 5′ exonuclease activity. Exonucleases are enzymes that can remove nucleotides from the ends of a DNA molecule, 3′ to 5′ exonucleases remove nucleotides from the 3′ end of a DNA molecule and therefore can remove the last nucleotide that was added during DNA replication. This is analogous to using the delete key to remove a letter that you have typed incorrectly before adding the correct one and continuing typing.
DNA polymerase requires a short double-stranded region with a free 3′ hydroxyl in order to start making a copy of the template; this ensures that DNA is synthesised in a controlled way. Initiation of DNA synthesis uses a small RNA primer (8–12 bases) made by the enzyme primase. DNA polymerase will then extend from the primer copying the template and synthesising the daughter DNA strand. This means that when DNA synthesis first starts each DNA molecule actually contains a small piece of RNA at its 5′ end. This RNA will ultimately be replaced with DNA, how this is done is discussed below.
The origin of replication and the replisome
A large multiprotein complex, called the replisome, is responsible for DNA replication. In prokaryotes, two replisomes form at a specific point on the chromosome called the Origin of Replication ( ori ). The DNA in this region will be opened up, ‘unzipped’ so that the replication machinery can gain access to single-stranded parental DNA, which will act as template for synthesis of the new daughter strands. The two replisomes then travel in opposite directions around the circular prokaryotic chromosome, each replisome forming a replication fork, a schematic representation of one replication fork is shown in Figure 6 .

A single replication fork showing the leading and lagging strands. The leading strand is synthesised continuously, reading the template 3′ to 5′, synthesising DNA in the 5′ to 3′ direction. The lagging strand is synthesised discontinuously, in short Okazaki fragments (1000 bases in prokaryotes and 100 bases in eukaryotes).
The replication fork
Within the replication fork, on the so-called leading strand, DNA polymerase moves 3′ to 5′ with respect to the template and synthesises DNA in the 5′ to 3′ direction as it moves in the same direction as the replication fork. Although overall the lagging strand is synthesised in the 3′ to 5′ direction, it is actually synthesised discontinuously in small segments called Okazaki fragments, which are synthesised 5′ to 3′ ( Figure 6 ). Each Okazaki fragment will be started with an RNA primer and is synthesised in the opposite direction to the movement of the replication fork. In prokaryotes, Okazaki fragments are 1000–2000 bases in length. In Figure 6 you will see that the DNA polymerase synthesising the Okazaki fragment will eventually reach the primer for the previous Okazaki fragment. When this happens the primer for the previous fragment is removed by a DNA polymerase using 5′ to 3′ exonuclease activity. DNA polymerase then replaces the missing nucleotides by adding them to the 3′ end of the last Okazaki fragment. When all the primer has been removed, there will be two DNA strands adjacent to each other but not joined by a phosphodiester bond, these two strands are joined together by the enzyme DNA ligase.
The replisome contains a number of other important proteins required for DNA replication. The double-stranded DNA needs to be separated, ‘unzipped’, by a helicase to generate the single-stranded DNA templates for DNA polymerase. As the replication fork moves along the helical DNA, the coils in the DNA in front of the fork become compressed so the DNA is described as being overwound; a topoisomerase is required to ‘relax’ it by remove the over-winding. Single-stranded binding proteins (SSBs) bind the lagging strand template to stabilise and protect the single-stranded DNA.
The two replication forks that form at the ori will move in opposite directions around the circular prokaryotic genome until they reach the terminator sequence, ter , which is on the opposite side of the genome compared with the ori , i.e. it is at 6 o’clock compared with 12 o’clock. This results in the complete replication of the genome. Once DNA replication has been completed a post-replication DNA repair process will correct errors that were not corrected by the proofreading activity of DNA polymerase. The fidelity of DNA replication is extremely high, resulting in an error rate of 1 mistake per 10 9 –10 10 nucleotides added.
DNA replication in eukaryotes
DNA replication is essentially the same in eukaryotes and prokaryotes. In both cases two replisomes form at an ori and generate two replication forks moving in opposite directions away from the origin. In each replication fork there are leading and lagging strands. There are two major differences. The first is that, due to the larger genome size, each chromosome has multiple origins of replication, so there will be a large number of replication forks on each chromosome.
The second difference is that, with the exception of mitochondrial DNA, eukaryotic chromosomes are linear and this results in an issue because of lagging strand synthesis. Replication of a linear chromosome results in shortening of one 5′ end of each daughter DNA molecule. This is because when the primer required for the last Okazaki fragment is removed, DNA polymerase cannot fill the gap ( Figure 7 A). Repeated rounds of DNA replication results in shorter and shorter DNA molecules. If this is not corrected, eukaryotes would have become extinct as their chromosomes get shorter with each generation. Eukaryotes have a mechanism to preserve the ends of chromosomes when it counts; that is in the gametes. The terminal ends of chromosomes, telomeres, contain a highly repeated sequence, for example, in humans the sequence TTAGGG is repeated in tandem 100 to over 1000-times. Repeated rounds of DNA replication will result in the shortening of these telomeric sequences that is the number of repeats will reduce. Telomerase, an RNA containing enzyme, can add additional copies of the repeat sequence to the 3′ end, replacing those lost during DNA replication (see Figure 7 ).

( A ) Following DNA replication and removal of the primer for the last Okazaki fragment of the lagging strand, there will be a region at the 3′ end that is not base paired, called a 3′ overhang. ( B ) Telomerase binds and uses the RNA it contains to act as a template to extend the 3′ overhang. This extends the 3′ end sufficiently for a new RNA primer to bind and the final Okazaki fragment to be made.
This actually extends the 3′ end of the telomere rather than extending the 5′ that is initially lost during DNA replication. The RNA sequence within telomerase is complementary to the 3′ telomeric sequence and so can bind and act as a template for synthesis of a short DNA sequence. Telomerase then moves along the newly synthesised strand and the process is repeated. Multiple rounds of elongation and translocation ultimately results in the 3′ end being extended so that it is long enough for it to act as template for synthesis of another Okazaki fragment, hence extending both strands of the telomere. Only germ cells and a few other actively dividing cells (e.g. haematopoietic cells) have sufficient levels of telomerase activity to counteract the loss of repeat sequences during DNA replication. At birth, telomeres are over 10000 base pairs in length and there are enough repeats to allow DNA replication and somatic cell division during the lifetime of the organism. If telomeres become too short this will trigger programmed cell death (a process called apoptosis). The lack of telomerase activity in somatic cells limits the number of cell divisions that can occur, and this is a ‘problem’ that needs to be overcome by cancer cells. Telomerase activity is reactivated in most cancers, allowing these cells to divide indefinitely and therefore this activity is a potential target for cancer therapies.
An understanding of DNA synthesis is central to many experimental approaches in molecular biosciences, it allows us to determine DNA sequences including that of the human genome, to analyse environmental samples to better understand the living world around us and to analyse minute biological samples from crime scenes to identify offenders. It is exploited in medicine, for example several drugs used to treat HIV infection or exposure are nucleoside analogues that inhibit DNA synthesis. Many chemotherapy agents used to treat cancer target DNA replication.
The genetic code and the concept of a gene
As we have seen in the previous two sections, the genetic material in a cell is made of DNA and can be copied and passed on to progeny through DNA replication allowing for inheritance of the information that it carries. A large proportion of the information on the DNA is first transcribed into mRNA and then translated into proteins. However there are some RNAs that are never translated into proteins and these have important functions too. Phrases like ‘it is in my genes’ or ‘in my DNA’ are used in common speech to mean to be an important part of who someone is.
The term gene was coined in the early 1900s to describe the basic unit of heredity. Genes were thought of as distinct loci arranged lineally on chromosomes. Breeding experiments with the fruitfly Drosophila supported this view and showed that if two genes are close together on a chromosome they are more likely to be inherited together. The observation that mutations in genes could give rise to altered phenotypes gave rise to the ‘one gene one polypeptide’ hypothesis. Once it became clear that genes were made of DNA, what is referred to as the central dogma of molecular biology was coined. This describes a two step process in which the genes on the DNA are transcribed into RNA and then translated into a sequence of amino acids that makes up a protein. The information flow is from DNA to RNA and then to protein ( Figure 8 ).

The arrows represent steps where DNA or RNA is being used as a template to direct the synthesis of another polymer, either RNA or protein.
However there are exceptions to this, firstly some viruses have RNA genomes and in some cases these are reverse transcribed into DNA before the genes can be expressed. The retrovirus HIV is an example of this. The other exception is that not all functional RNAs are translated into proteins (see non-coding RNAs below).
The genetic code
The genetic code is the set of rules used by living cells to translate the information encoded within genetic material into proteins. When DNA and RNA were first discovered, the relative simplicity of nucleic acids led many scientists to doubt that it carried the genetic information. DNA only has four different kinds of bases; the question was how it could code for 20 amino acids. If there were a 1:1 correlation between bases and amino acids DNA could only encode four amino acids. Pairs of bases would give 16 possible combinations which is still not enough. However if you consider a triplet code you have 64 possibilities, which is more than enough. This is the code that we are familiar with where each codon, a sequence of three nucleotides, specifies a particular amino acid. This triplet code still did not seem logical because now you have far more codons than you need. There are some other important questions about the genetic code too; are the spare codons used? Is the code overlapping? And is it continuous or are there spacers indicating the end of each codon?
Table 1 shows the genetic code as we now understand it. It is written as RNA with a U rather than a T because it is RNA that cells translate into amino acids. The code is said to be redundant or degenerate because a single amino acid is often coded for by more than one codon. In most cases it is the third nucleotide in the codon that differs; this is often referred to as the degenerate position.
Evidence for the triplet code
The experiments that allowed scientists to decipher the genetic code were carried out long before we were able to determine the sequence of DNA. While it was possible at that time to determine the proportions of each different amino acid in a protein, it was not yet possible to work out the order in which they occurred. Francis Crick and Sydney Brenner answered some key questions with an experiment using mutants of a virus that infects bacteria called bacteriophage. The normal or wild-type phage will infect E. coli and grow. Crick and Brenner investigated mutants that would not grow on some strains of E. coli .
Mutants which are insertions or deletions cause what are called frameshifts. Inserting a single adenine base into the DNA sequence not only changes the amino acid at the position of the insertion but all subsequent amino acids translated from that sequence (Compare Figures 9 A and B); the reading frame has been shifted by one base and it results in a protein that is non-functional. However if you insert three nucleotides you often get a wild-type or near wild-type phenotype. This is because you have inserted a whole triplet codon, you will get one or two amino acids that were not in the original sequence but the reading frame is not shifted ( Figure 9 C) and the rest of the sequence is normal.

( A ) Wild-type sequence, ( B ) a single base insertion (shown in red) causes a frameshift so all subsequent amino acids are different from the wild-type, ( C ) insertion of three base pairs (shown in red) causes two incorrect amino acids to be incorporated into the protein but there is no frameshift so the rest of the protein has the wild-type sequence.
Crick and Brenner were looking for what they called suppressor mutations that would rescue the mutant and allow it to grow normally. They showed that their suppressor mutants did not simply reverse the original mutation; they often added or subtracted one or more bases. They worked out that if you insert or delete one, two or four nucleotides then you see a mutant phenotype. However, if you insert or delete three nucleotides, this has little or no effect. This was strong supporting evidence for a triplet code. This is also evidence for a redundant code where the same amino acid can be coded in more than one way. If the code were non-redundant there would be 20 codons that code for amino acids and 44 that are ‘nonsense’ codons. In this case inserting three nucleotides would be most likely to introduce a nonsense codon and not restore the wild-type. Crick and Brenner proposed correctly that the genetic code is read from a fixed starting point and the bases are read in groups of three.
Cracking the code
At about the same time two American scientists Marshall Nirenberg and Heinrich Matthaei had developed a cell-free system which could synthesise proteins in a test tube when provided with an RNA molecule. They showed that when provided with an artificial RNA chain composed only of uracil (polyuracil) the system made a polypeptide composed entirely of phenyalanine residues. They now had a tool that they could use to crack the genetic code. RNA composed of cytosine (C) residues directed the synthesis of polyproline and RNA composed of adenosine (A) made polylysine. Experiments with combinations of nucleotides demonstrated that, for example, if you make RNA from A and C you produce proteins containing only six amino acids: asparagine, glutamine, histidine, lysine, proline and threonine. There are eight possible triplet codons that can be made from A and C, two of these we know encode proline and cysteine. The remaining four amino acids must be encoded by other combinations of A and C. This of course provides additional evidence for the redundancy of the genetic code.
These experiments using RNA molecules composed of random combinations of two or three bases were not enough to fully crack the genetic code. The use of chemically synthesised RNA molecules of known repeating sequence added some more important information. For example a synthetic RNA of alternating A and G residues (AGAGAGAGAG…) can be read as two alternating codons CAC and ACA. It encodes a protein of alternating histidine and threonine residues.
In the last section, we will discuss how tRNAs and ribosomes decode the genetic code and synthesise proteins. The final detail of the genetic code was determined by a technique using ribosome-bound tRNAs. Pieces of RNA as short as a single codon will bind to ribosomes and if amino acids attached to tRNA are added they will associate with the complementary RNA. If you then filter the solution you trap only the tRNAs that are bound to the ribosome, these are the ones specified by the codon in your RNA.
Start and stop codons
Of the 64 possible codons, 61 encode amino acids. The three remaining codons: UAA, UAG and UGA do not code for an amino acid, they are sometimes called nonsense codons. They are stop codons; when the ribosome encounters these protein synthesis stops. The AUG codon encodes the amino acid methionine but it is also the most common start codon. As you will see in the last section, the first residue in eukaryotic proteins is always a methionine and in prokaryotes it is a modified amino acid N-Formylmethionine.
Expanding the genetic code
Nature uses a small set of amino acids to make proteins, however if we were able to engineer cells that could use a wider range of building blocks with different physical and chemical properties it would be possible to make novel materials some of which could have useful therapeutic properties; this is one of the aims of synthetic biology. To do this successfully we need to reprogramme the genetic code and to engineer the translation machinery (see later section) to use these new combinations. Some progress has been made, for example in using both the UAG stop codon and the AAG codon for arginine to code for amino acids not normally found in proteins.
Current concept of the gene
Once the genetic code was cracked it was clear that a gene is a sequence of bases on a DNA molecule that codes for a sequence of amino acids in a polypeptide chain or for an RNA molecule with a specific function. The availability of DNA sequences (see ‘Recombinant DNA Technology and DNA Sequencing’ in this issue of Essays in Biochemistry ) of individual genes made it possible to look for patterns characteristic of genes. A gene that codes for a protein has a start codon followed by a series of codons that encode the amino acid sequence and then a stop codon; this is called an open reading frame.
Whole genome sequencing has provided biological data on an unprecedented scale. The need to analyse sequence data has led to the development of the field of bioinformatics; the analysis of these data to answer biological questions. One key concept used in bioinformatics is that of homology. Two organisms that have a common ancestor are said to be homologous and the same can be said of a structure or of a gene. For example limbs with five digits (the pentadactyl limb) are found not only in humans and other mammals but also in birds, reptiles and amphibians. The limbs are homologous, and this is evidence of a common evolutionary ancestor of all of these groups of animals. The same is true of genes. All vertebrates have red blood cells that contain haemoglobin, adult human haemoglobin is made from two α and two β globin molecules. The DNA sequence of the genes that encode globin molecules in vertebrates are all similar to each other and you can estimate how long ago two animals shared a common ancestor by looking at how similar their globin genes are. This principle can also be used to find genes in a new piece of DNA sequence; if there is a section of sequence that is similar to a known gene then it is likely to encode a homologous gene.
A gene is more than just the sequence that encodes the protein; it also includes sequences involved in regulation of gene expression such as promoter sequences that define where transcription starts and are the sites where proteins involved in transcription bind to the DNA. In bacteria, almost all genes are a single uninterrupted sequence of DNA. In eukaryotes the situation is more complicated because the coding region is usually interrupted by introns. The primary transcript is referred to as precursor or pre-mRNA, this contains both exons and introns. The introns are removed when the pre-mRNA is processed before it leaves the nucleus ( Figure 10 ) leaving the exons which are spliced together to make the mature mRNA. Eukaryotic mRNAs have a 5′ cap which is a methylated guanosine nucleotide added to the 5′ end of the mRNA by an unusual 5′ to 5′ linkage; this is important in initiating translation. At the 3′ end is the poly A tail, this is a chain of between 100 and 250 adenine residues added to the mRNA to increase its stability. Analysis of the human genome sequence suggests that there are approximately 20000–25000 protein-coding genes, however there are far more different proteins. This is because many genes are capable of encoding several variants of a protein. Alternative splicing allows for different combinations of exons to be included in the mature mRNA and genes can also have several alternative promoters and alternative poly A sites. It is thought that 95% of human genes are alternatively spliced.

The DNA includes an untranslated region at both the 5′ and 3′ ends as well as introns and exons. The codon where translation starts (green) and the stop codon (red) are shown. The DNA is transcribed into mRNA and is processed by addition of the 5′ cap, splicing out the introns and addition of the poly A tail. This mature mRNA is exported from the nucleus into the cytoplasm.
Non-coding RNAs
Only approximately 1.2% of the human genome codes for protein. However, if you compare the genomes of the human, the mouse and the dog you can see that much more of the genome is under what is called negative selection since the species diverged. Negative selection means that mutations which are disadvantageous are selected against. This suggests that more than just the protein coding regions affect the fitness of the organism carrying the DNA. Some of these are DNA sequences that are important in controlling gene expression (next section). However systematic screens are revealing large numbers of RNA transcripts that are processed but do not encode proteins. The most well-known are transfer RNAs and ribosomal RNAs both of which as we will see in a later section are fundamental to protein synthesis. However we are beginning to understand that there are other non-coding RNAs that carry out important cellular processes.
Two types of non-coding RNA, small inhibitory RNAs (siRNAs) and microRNAs (miRNAs) have a role in reducing gene expression after the mRNA has been transcribed from the DNA. They work by targeting a protein complex called RISC to specific mRNAs which it then degrades. Expression of the gene is specifically knocked out or reduced and the phenotypic effect of this can then be observed. Another group of non-coding RNAs play an important role in increasing the stability and correct folding of ribosomal RNAs. This process takes place in a compartment within the nucleus called the nucleolus; the RNAs are called small nucleolar RNAs (snoRNAs). These are mostly generated from intron RNA after it has been spliced out of the precursor mRNA and they function in association with proteins.
Modern concept of a gene
The modern concept of the gene has to take into account all of the complexity of mRNA processing including alternative splicing, regulatory sequences and polyadenylation sites as well as the plethora of non-coding RNAs. A definition of a gene that takes these factors into account would be that a gene codes for one or more transcripts that can function as an RNA or can be translated into one or more proteins.
Transcription
We have seen that a gene can encode either an RNA product or a protein sequence. The production of both requires the gene to be transcribed into RNA, either because the RNA is the final product or because the RNA will need to act as template for protein synthesis. RNA synthesis is very similar in prokaryotes and eukaryotes, being catalysed by the enzyme RNA Polymerase. However, of the processes discussed in this article it is arguably the one that differs most between prokaryotes and eukaryotes. One difference is that in eukaryotes the whole process needs to occur in a chromatin context, so access to the DNA template is limited. Regulation of gene expression is a major facilitator of cell differentiation, homoeostasis and speciation. Different cell types turn on transcription of different genes giving rise to their differentiated phenotypes. If we look at mammals as an example of speciation, they all have roughly the same gene content; it is how transcription is regulated that has changed as mammals have evolved. For example, if you compare humans and mice, the important changes to the human and mouse genome sequence that have occurred since they diverged from a common ancestor, are predominantly in the sequences that control transcription rather than in protein coding sequences.
RNA polymerase
DNA-dependent RNA polymerases are responsible for transcription of DNA into RNA. Like DNA Polymerase, RNA polymerase requires a DNA template and nucleoside triphosphate precursors. RNA polymerase does not require a primer. During RNA synthesis, the base within the incoming nucleoside triphosphate pairs with the base on the DNA template, a phosphodiester bond is formed, and pyrophosphate is released. RNA polymerase synthesises RNA in the 5′ to 3′ direction, because it can only add nucleotides on to the 3′ end of the chain. During transcription only one DNA strand is transcribed into RNA.
Gene transcription
When a gene is transcribed, RNA polymerase will bind upstream from the start of the gene, it will unwind almost two turns of the DNA helix to form a transcription bubble, it will add nucleotides on to the growing RNA chain, the last 12 nucleotides to be added to the RNA chain will base pair with the DNA template, forming a DNA–RNA heteroduplex ( Figure 11 ).

As each nucleotide is added to the growing chain, the transcription bubble and the heteroduplex moves with respect to the DNA template. So, as RNA polymerase synthesises RNA, there is unwinding of the DNA template in front of the site of synthesis and rewinding of DNA once RNA polymerase has passed through. Once RNA polymerase has transcribed the gene, transcription will terminate. For some genes, transcription termination is signalled by a particular sequence within the DNA, a terminator sequence, which RNA polymerase recognises. In some cases, RNA polymerase requires the help of other protein factors to recognise the terminator sequence. Finally, many eukaryotic genes do not contain a specific terminator sequence; instead, termination of transcription is linked to other events, for example cleavage of the RNA prior to addition of the polyA tail. Termination of transcription, leads to the dissociation of RNA polymerase from the DNA template and release of the RNA product. In prokaryotes, mRNA does not need processing before it can be translated, in fact, as will be discussed below, mRNA is translated as it is being made. However, the initial transcript in eukaryotes does need to be processed to produce a functional mRNA that can be exported to the cytoplasm for translation.
Control of transcription in prokaryotes
At many genes in prokaryotes, RNA polymerase can bind to the gene and initiate transcription without other protein factors. However, for most prokaryotic genes, the binding of RNA polymerase to the gene is controlled by transcription factors to ensure the correct genes are transcribed at the correct level within the cell. Upstream from the transcription start there will be a ‘promoter’ which contains specific DNA sequences that are recognised by RNA polymerase and transcription factors. Each gene will have a different promoter sequence and can be controlled by different transcription factors. A good example of this type of promoter is the promoter that controls the lac operon in E. coli ( Figure 12 ). Transcription factors that up-regulate transcription are called activators and those that down-regulate transcription are called repressors. In this example RNA polymerase on its own can bind the promoter and drive low levels of transcription. If the repressor binds it will stop all transcription and would override RNA polymerase and the activator. In the absence of the repressor, if the activator is present then it can drive high levels of transcription.

The different binding sites for transcription factors are shown on the DNA; ABS, activator binding site, RBS, repressor binding site. The left-hand panel indicates the presence of lactose and/or glucose in the environment, the right-hand panel indicates transcription levels.
The lac operon codes for genes required to use lactose and needs to be controlled in response to glucose and lactose concentrations. A repressor protein is responsible for responding to lactose concentration and an activator is responsible for responding to glucose ( Figure 12 ).
In the absence of lactose, the lac operon is kept in an off state by the repressor protein binding to the promoter and stopping transcription. If lactose is present in the cell, it will bind to the repressor and this stops the repressor binding the promoter, RNA polymerase can bind and drive low levels of transcription. If the cell is starved of glucose, the activator is turned on and this binds the promoter and helps RNA polymerase to initiate transcription, resulting in high rates of transcription.
In the examples above, RNA polymerase on its own drives low levels of transcription. This might not be the case for all promoters, at some promoters RNA polymerase may not be able to bind and drive transcription without an activator protein. At other promoters RNA polymerase on its own will be able to drive high levels of transcription and a repressor protein would be needed to turn off transcription.
Control of transcription in eukaryotes
Control of transcription in eukaryotes has to occur on a chromosome which is condensed into chromatin ( Figure 13 ). In addition, transcription requires the assembly of a large multiprotein complex at the gene. This complex will contain RNA polymerase and several other general transcription factors (GTFs). The core promoter is a region that overlaps the transcription start and is the binding site for RNA Polymerase and the GTFs. In addition, there will be further control sequences, enhancers, that can be just upstream or several 1000 base pairs away from the core promoter. In the absence of activator proteins the chromatin structure will stop RNA polymerase and the GTFs binding to the core promoter. Here histone proteins act as generic repressors of transcription. In order for a transcription to be turned on activators will bind the enhancers and recruit co-activators which open up the chromatin structure and ensure the core promoter is not blocked by histone proteins. The activators and co-activators will then assemble RNA polymerase and the GTF at the core promoter and drive transcription initiation. Transcription factors will also ensure the chromatin structure across the whole gene is in a conformation that is suitable for transcription.

( A ) When a gene is in a silent state the surrounding DNA will be in condensed chromatin and the histones will epigenetic modifications which facilitate gene repression (red spheres). ( B ) A gene that is being transcribed will have activators bound to enhancer sequences, the activators recruit co-activators that acetylate the histone and add other epigenetic modifications that facilitate gene transcription (green spheres). The activator and co-activators will recruit RNA Polymerase and the GTFs to the core promoter.
Repressors are not normally required to block assembly of transcription complex at the core promoter, however, they are important in the regulatory patterns needed in complex multicellular organisms. Eukaryotes have repressor proteins which can block the action of a specific activator and ensure the activator is only active when required. Repressors can work in a number of ways including binding to DNA and blocking the binding of the activator to the DNA, stopping the activator interacting with other proteins required for transcription or by binding to the activator and keeping the activator in the cytoplasm.
Epigenetics
As discussed above transcription initiation in eukaryotes requires the opening up of the chromatin structure. This is facilitated by co-activator proteins that can move the relative position of the nucleosomes ( Figure 4 ) with respect to the DNA and hence make certain regions of the DNA more accessible. They can also add chemical tags to both the histone proteins and DNA ( Figure 13 ). These epigenetic modifications can affect whether a gene or genomic region is available for transcription or is transcriptionally silenced. Histones are acylated by enzymes which transfer an acetyl functional group to from acetyl-coenzyme A to lysine residues in the histone protein. This is linked to activation of transcription because it reduces the positive charge on histones and therefore reduces their affinity for the negatively charged DNA. Acetylation can also act as a tag that is recognised by other proteins that drive gene transcription. This modification of the DNA is described as epigenetic because it affects gene expression rather than the genetic code itself. Conversely some repressor proteins will recruit co-repressors that deacetylate histones, increasing their affinity for DNA causing the chromatin to be highly condensed and leading to transcriptional silencing. Methylation of lysine residues is another epigenetic tag, a single lysine residue can have 1, 2 or 3 methyl groups added. Unlike acetylation, methylation of lysine residues does not change the positive charge. The consequences of histone methylation are more complex because depending on which lysine residue is methylated and the level of methylation, the tag may mark that region of the genome for transcription activation or repression.
DNA methylation is another important epigenetic modification which leads to transcriptional silencing of the genomic region that has been methylated. During differentiation in the developing embryo whole regions of the genome will be methylated and therefore transcriptionally silenced. The DNA methylation patterns are maintained during cell division and future generations of that cell.
Analysing transcription on a global scale
For many years individual scientists would study the transcriptional regulation of their ‘favourite’ gene and so we gained an understanding of how individual genes were regulated in response to different development or environmental signals, for example the control of the lac operon in response to lactose and glucose. In the last 15 years, many techniques have been developed to allow us to study transcriptional control of genes within a cell. Using techniques such as ‘RNA-Seq’, we can isolate the total RNA from a cell and use high-throughput sequencing to catalogue the level of transcription of all genes. In the case of eukaryotes this will also show how they have been spliced. It is also possible to analyse the binding of transcription factors and study epigenetic changes within histone proteins across the genome using techniques such as ChIP-Seq. So, combining techniques such as RNA-Seq and ChIP-Seq we can determine when and where a protein factor is bound to DNA and study epigenetic changes in a particular cell type and the consequences in terms of gene transcription. In combination these techniques give a detailed picture of the factors that affect transcription; this has been used, for example to look at differences between cancer cells and normal cells from the same patient.
Transcription and disease
Transcription factors and promoters play major roles in health and disease, below are just a few examples to give an idea of their role in health and disease.
- The transcription factor p53 is a tumour suppressor protein, it guards against cancer and some human cancers have mutations that knock out p53 function.
- The drug Tamoxifen used in the treatment of breast cancer binds the oestrogen receptor inhibiting its function. The oestrogen receptor is a transcription factor that turns on the transcription of genes in response to oestrogen.
- Rett Syndrome is a neurodevelopmental disorder that affects approximately 1 in 15000 female births. It is due to mutations in a transcription factor that would normally repress transcription of specific genes, the mutations lead to inappropriate transcription of these genes.
- Cocaine use results in changes in expression of many genes, this can include epigenetic changes within genes involved in cognition and brain function. These epigenetic changes can be inherited and there is evidence that cocaine use by a father can result in epigenetic changes that result in male, but not female, offspring being cocaine resistant.
Translation of RNA into proteins
The key player in protein synthesis is the ribosome, a complex structure composed of RNA and proteins. The ribosome provides a framework that ensures that the mRNA and tRNA are correctly positioned enabling the deciphering of the genetic code. There are many other proteins that are important in protein synthesis; some of these are part of the ribosome and some are again correctly positioned by the framework of the ribosome. As we will see, the small subunit ribosomal RNA is a ribozyme; an RNA molecule with catalytic properties similar to those of enzymes. Ribosomal RNA can form a peptide bond between two amino acids.
Transfer RNA
The other nucleic acid that you need for protein synthesis is the tRNA. The tRNA molecule is single stranded and folds up into a characteristic structure by base pairing ( Figure 14 ). These act as adaptor molecules, each has an anticodon for a specific mRNA codon and each carries the amino acid specified by that codon. The anticodon has a complementary sequence to the codon on the mRNA.

( A ) Tertiary structure of the phenylalanine tRNA from yeast showing the anticodon (grey), the acceptor stem (violet) with the nucleotides CAA at the 3′ OH end (yellow). Image modified from ‘TRNA-Phe yeast’ Yikrazuul (licensed under CC BY-SA 3.0). ( B ) Clover leaf representation of the secondary structure of tRNA.
The enzymes which attach amino acids to tRNAs are called aminoacyl tRNA synthetases; they recognise a specific amino acid and the corresponding tRNA. The reaction also requires ATP, it is carried out in two steps:

In the first step the enzyme hydrolyses ATP releasing pyrophosphate (PP) and in the second it attaches the amino acid to the 3′ hydroxyl of the tRNA. Aminoacyl tRNA synthetase enzymes are highly specific, they recognise specific amino acids and will only attach them to the correct tRNA. This ensures correct coupling of amino acids and tRNA molecules which is just as important in ensuring the fidelity of protein synthesis as the matching of the anticodon to the codon by the ribosome. In addition this step is said to activate the aminoacyl tRNA as it not only produces the correct substrate for the ribosome but also provides much of the energy required for peptide bond formation during protein synthesis.
Structure of the ribosome
All living things contain ribosomes. The ribosomes in bacteria are slightly smaller than those found in eukaryotic cells ( Table 2 ) but the overall structure and the way in which they work are essentially the same. The 2009 Nobel Prize for Chemistry was awarded to three scientists, Ada Yonath, Thomas Steitz and Venkatraman Ramakrishnan, who used X-ray crystallography to solve the three-dimensional structure of the bacterial ribosome. The ribosome is composed of two subunits, the small subunit which reads the messenger RNA and the large subunit which forms the bonds between amino acids, adding them to the growing polypeptide chain. There are three important binding sites for tRNAs in the ribosome which are at the interface between the two subunits and only formed when the two subunits come together. These sites are shown on the image in Figure 15 , they are referred to as the acceptor or aminoacyl (A) site, the peptidyl (P) site where the peptide bond between amino acids is formed and the exit (E) site from which spent tRNAs leave the ribosome.

In ( A ) the new tRNA is delivered to the ribosome by elongation factor EF-Tu (purple). In ( B ) the amino acid on the incoming tRNA is brought close to the amino acid on the tRNA in the peptidly site to facilitate peptide bond formation (bright green) (Adapted from Goodsell 2010 , licensed under CC-BY-4.0 licence).
Bacterial ribosome | Eukaryotic ribosome | |
---|---|---|
Size | 70S , 2.3–2.6 MDa | 80S, 3.3–4.5 MDa |
Small subunit | 30S | 40S |
Approximately 20 proteins | 32 proteins | |
16S rRNA, ∼1600 nucleotides | 18S rRNA | |
Large subunit | 70S | 60S |
Approximately 33 proteins | 79–80 proteins | |
23S rRNA, ∼2900 nucleotides | 25S rRNA | |
5S rRNA, ∼120 nucleotides | 5.8S & 5S |
In addition to the ribosome, the mRNA and tRNA, there are a number of small proteins that are not part of the structure of the ribosome, but are required for protein synthesis: initiation factors, elongation factors and termination factors. The importance of these factors is illustrated by the inherited condition Vanishing White Matter Disease (VWM). This serious neurodegenerative disease which results in lesions in the white matter in the brain is due to mutations in one of the initiation factors.
Protein synthesis
During protein synthesis the ribosome brings together the amino acid charged tRNA and the mRNA, the codon and anticodon are matched and the amino acids are joined together in the correct sequence. There are three phases to this process: initiation where the ribosome assembles on the mRNA, elongation where the triplet code is read and amino acids are added to the growing peptide chain and termination where protein synthesis stops.
A complex of proteins called the cap-binding complex bind to the 5′ cap of the mRNA ( Figure 10 ) in the nucleus. The mRNA is then exported to the cytoplasm where it recruits initiation factors, tRNA charged with a methionine and the small (40S) ribosomal subunit. Initiation factors also bind and the small subunit scans along the 5′ untranslated region of the mRNA until it encounters the first AUG start codon (Figure 16A). This is recognised by the anticodon codon of the initiator tRNA, the large subunit then docks to give the translation complex. The 80S ribosome with the tRNA charged with methionine at the P site is now ready to accept the next tRNA ( Figure 16 B).

( A ) During initiation, the mRNA recruits a tRNA charged with a methionine and the small ribosomal subunit, ( B ) the large subunit then docks to give the translation complex, ( C ) a tRNA with an amino acid attached enters the A site, ( D ) the peptide bond is formed between the amino acid in the P site and the one in the A site. The effect is that the growing peptide chain is transferred to the incoming aminoacyl tRNA in the A site leaving an empty tRNA in the P site. ( E ) Finally, everything moves along the mRNA by one codon in a process called translocation so the peptidyl tRNA with the growing peptide chain attached moves to the P site and the spent tRNA to the E site from where it leaves the ribosome. ( F ) When a stop codon is in the A site, a termination or release factor enters the A site, ( G ) the peptide is released from the ribosome and ( H ) the two subunits of the ribosome disassociate and are recycled.
With initiation complete, the mRNA is in the correct reading frame with the A site empty and the next codon exposed. In the elongation phase an aminoacyl tRNA, one charged with an amino acid, is brought to the ribosome in a complex with an elongation factor and enters the A site. If the anticodon it carries is complementary to the exposed codon it is correctly positioned in the acceptor site and GTP is hydrolysed on the elongation factor ( Figure 16 C). A peptide bond ( Figure 17 ) is then formed between the C terminus of the amino acid in the P site and the N terminus of the amino acid in the A site, this reaction is catalysed in the peptidyl transfer centre of the large subunit of the ribosome. The effect is that the growing peptide chain is transferred to the incoming aminoacyl tRNA in the A site leaving an empty or spent tRNA in the P site ( Figure 16 D). Finally, the peptidyl tRNA with the growing peptide chain attached moves to the P site. This step is called translocation and the energy being provided by hydrolysis of GTP by the elongation factor EF-G. The spent tRNA moves to the exit site from where it can leave the ribosome. The mRNA moves so that the next codon is exposed in the A site ( Figure 16 E) ready to accept a new aminoacyl-tRNA charged with another amino acid. During the elongation phase the ribosome cycles through this process, adding amino acids to the growing peptide chain until a stop codon is exposed in the A site. The new protein emerges from the ribosome through an exit tunnel in the large subunit.

( A ) Amino acids consist of a carbon atom with an amine group (the N terminus), a carboxylic acid group (the C terminus) and a variable R group. The simplest R group is a methyl group giving the amino acid alanine. ( B ) When two amino acids are joined together a peptide bond is formed between the N terminus of one amino acid and the C terminus of another. This is a condensation reaction releasing one molecule of water.
Termination
The stop codon is not decoded by being recognised by an anticodon on a tRNA. Instead it is detected by proteins called termination or release factors. In eukaryotes there is a single release factor (RF1) that recognises all three stop codons enters the A site ( Figure 16 F). The ester bond linking the peptide chain to the tRNA in the P site is broken and the peptide is released from the ribosome ( Figure 16 G) The two subunits of the ribosome disassociate and are recycled ( Figure 16 H).
The structure and function of ribosomes are highly conserved with a large core of structurally conserved proteins and rRNAs found in both eukaryotic and prokaryotic ribosomes. However, there are some differences both in the rRNAs and in some of the additional proteins involved in translation ( Table 2 ). The elongation phase is highly conserved but there are important differences in how protein synthesis is initiated. Bacterial mRNAs have a specific sequence called the ribosome binding site or Shine–Dalgarno sequence. In order to ensure that the mRNA is correctly positioned in the ribosome the Shine–Dalgarno sequence binds to a complementary sequence of the 16S rRNA in the small subunit. In bacteria the initiator tRNA is charged with a modified amino acid N-Formylmethionine.
Differences between the structure of bacterial and eukaryotic ribosomes can be exploited by antibiotics which are selective in that they affect protein synthesis in bacteria but not in mammalian cells. Macrolide antibiotics like erythromycin, block the exit tunnel in the large subunit of bacterial ribosomes and halt protein synthesis. The exit tunnel in eukaryotic ribosomes is slightly narrower which means that eukaryotic ribosomes are not affected. Streptomycin, an important antibiotic in the treatment of tuberculosis binds to the 16S of bacterial ribosomes. This distorts the structure of the decoding site and results in misreading of the mRNA.
Polyribosome
Protein synthesis can proceed very quickly, particularly in rapidly growing cells or those that are differentiating. In bacteria between 15 and 20, new peptide bonds can be formed per second. In eukaryotes it is slower, more like five peptide bonds per second. A small human protein like insulin would take only 10 seconds to make whereas the largest human protein titin, which is found in human muscle cells, takes about an hour and a half per molecule. One of the mechanisms that ensures that protein synthesis is carried out efficiently is the polyribosome. As soon as one ribosome has started translation another ribosome binds to initiate synthesis of another protein copy. This gives rise to polyribosomes or polysomes which can be seen by electron microscopy. Recent cryo-EM images show that ribosomes can be arranged very closely on the mRNA with the mRNA entry and exit channels aligned to allow the smooth passage of mRNA between them ( Figure 18 ). Sometimes these polyribosomes can form circular structures so that, as soon as the ribosome has finished synthesis of one polypeptide it can rebind the same mRNA molecule and start synthesis of another copy of the protein.

Cyro-electron micrograph reconstruction of eukaryotic polyribosome. Reprinted from ( Myasnikov 2014 ) by permission.
Closing remarks
The study of nucleic acids, from their first identification as the genetic material is littered with landmarks in molecular biosciences, many of them marked with Nobel Prizes. Since Watson and Crick proposed their structure of DNA our knowledge about DNA and how it works has expanded almost exponentially. The topics introduced in this article are important topics covered in all bioscience programmes; understanding them is key to all areas of biosciences from evolution and animal diversity to health and disease. Recent developments in the techniques that we can use to study DNA, often in living cells means that new and exciting developments in our understanding of the way nucleic acids work are occurring all the time. Given the scope of this article we have barely scratched the surface of the topic, however, the reader can find more detail from the articles in the bibliography below and even more detail from a few minutes searching on the internet.
Abbreviations
DNA | deoxyribonucleic acid |
GTF | general transcription factor |
ori | origin of replication |
RNA | ribonucleic acid |
RISC | RNA-induced silencing complex |
Competing interests
The authors declare that there are no competing interests associated with the manuscript.
Recommended reading and key publications Nobel lectures
- Blackburn E.H. (2010) Telomeres and Telomerase: The Means to the End (Nobel Lecture) 49 , Int. Ed., pp. 7405–7421, Angewandte Chemie [ PubMed ] [ Google Scholar ]
- Ehrenberg M. (2009) Scientific Background on the Nobel Prize in Chemistry 2009 Structure and Function of the Ribosome , The Royal Swedish Academy of Sciences, https://www.nobelprize.org/uploads/2018/06/advanced-chemistryprize2009.pdf [ Google Scholar ]
- Kornberg R.D. (2007) The Molecular Basis of Eukaryotic Transcription (Nobel Lecture) 32 , Int. Ed., pp. 12955–12961, Angewandte Chemie [ PubMed ] [ Google Scholar ]
Review articles
- Afonina Z.A. and Shirokov V.A. (2018) Three dimensional organization of polyribosomes–a modern approach . Biochemistry (Moscow) 83 , S48–S55 10.1134/S0006297918140055 [ PubMed ] [ CrossRef ] [ Google Scholar ]
- Gerstein M.B, Bruce C., Rozowsky J.S., Zheng D, Du J., Korbel J.O.. et al. (2007) What is a gene, post-ENCODE? History and updated definition . Genome Res. 17 , 669–681 10.1101/gr.6339607 [ PubMed ] [ CrossRef ] [ Google Scholar ]
- Kruglyak L. and Stern D.L. (2007) An embarrassment of switches . Science 317 , 758–759 10.1126/science.1146921 [ PubMed ] [ CrossRef ] [ Google Scholar ]
- Minchin S.D. and Busby S.J.W. (2013) Transcription factors . In Brenner’s Encyclopedia of Genetics (Maloy S. and Hughes K., eds), Elsevier, U.S.A. [ Google Scholar ]
- Roberts M. (2019) Recombinant DNA technology and DNA sequencing . Essays Biochem. 63 , 10.1042/EBC20180039 [ PubMed ] [ CrossRef ] [ Google Scholar ]
- Roeder R.G. (2003) The eukaryotic transcriptional machinery: complexities and mechanisms unforeseen . Nat. Med. 9 , 1239–1244 10.1038/nm938 [ PubMed ] [ CrossRef ] [ Google Scholar ]
Historical perspectives
- Dahm R. (2008) Discovering DNA: Friedrich Miescher and the early years of nucleic acid research . Hum. Genet. 122 , 565–581 10.1007/s00439-007-0433-0 [ PubMed ] [ CrossRef ] [ Google Scholar ]
- McCarty M. (2003) Discovering genes are made of DNA . Nature 421 , 406 10.1038/nature01398 [ PubMed ] [ CrossRef ] [ Google Scholar ]
- Maddox B. (2003) The double helix and the “wronged heroine” . Nature 421 , 407–408 [ PubMed ] [ Google Scholar ]
- Kemp M. (2003) The Mona Lisa of modern science . Nature 421 , 416–420 10.1038/nature01403 [ PubMed ] [ CrossRef ] [ Google Scholar ]
Original research papers
- Crick F.H.C., Barnett L., Brenner S. and Watts-Tobin R.J. (1961) General nature of the genetic code for proteins . Nature 192 , 1227–1232 10.1038/1921227a0 [ PubMed ] [ CrossRef ] [ Google Scholar ]
- Franklin R.E. and Gosling R.G. (1953) Molecular configuration in sodium thymonucleate . Nature 171 , 740–741 10.1038/171740a0 [ PubMed ] [ CrossRef ] [ Google Scholar ]
- Meselson M. and Stahl F.W. (1958) The replication of DNA in Escherichia coli . Proc. Natl. Acad. Sci. U.S.A. 44 , 671–682 10.1073/pnas.44.7.671 [ PMC free article ] [ PubMed ] [ CrossRef ] [ Google Scholar ]
- Watson J. and Crick F. (1953) Molecular structure of nucleic acid. A structure for deoxyribose nucleic acid . Nature 171 , 737–738 10.1038/171737a0 [ PubMed ] [ CrossRef ] [ Google Scholar ]
Citations for figures
- Goodsell D. (2010) Molecule of the month: ribosome . https://pdb101.rcsb.org/motm/121 [ Google Scholar ]
- Myasnikov A.G. (2014) The molecular structure of the left-handed supra-molecular helix of eukaryotic polyribosomes . Nat. Commun. 5 , 5294 10.1038/ncomms6294 [ PubMed ] [ CrossRef ] [ Google Scholar ]
- Yikrazuul X.X. (2010) tRNA-Phe yeast . https://commons.wikimedia.org/wiki/File:TRNA-Phe_yeast_1ehz.png [ Google Scholar ]

An official website of the United States government
The .gov means it’s official. Federal government websites often end in .gov or .mil. Before sharing sensitive information, make sure you’re on a federal government site.
The site is secure. The https:// ensures that you are connecting to the official website and that any information you provide is encrypted and transmitted securely.
- Publications
- Account settings
- My Bibliography
- Collections
- Citation manager
Save citation to file
Email citation, add to collections.
- Create a new collection
- Add to an existing collection
Add to My Bibliography
Your saved search, create a file for external citation management software, your rss feed.
- Search in PubMed
- Search in NLM Catalog
- Add to Search
The 2022 Nucleic Acids Research database issue and the online molecular biology database collection
Affiliations.
- 1 Institute of Systems, Molecular and Integrative Biology, University of Liverpool, Crown Street, Liverpool L69 7ZB, UK.
- 2 Institut Curie, 25 rue d'Ulm, 75005 Paris, France.
- PMID: 34986604
- PMCID: PMC8728296
- DOI: 10.1093/nar/gkab1195
The 2022 Nucleic Acids Research Database Issue contains 185 papers, including 87 papers reporting on new databases and 85 updates from resources previously published in the Issue. Thirteen additional manuscripts provide updates on databases most recently published elsewhere. Seven new databases focus specifically on COVID-19 and SARS-CoV-2, including SCoV2-MD, the first of the Issue's Breakthrough Articles. Major nucleic acid databases reporting updates include MODOMICS, JASPAR and miRTarBase. The AlphaFold Protein Structure Database, described in the second Breakthrough Article, is the stand-out in the protein section, where the Human Proteoform Atlas and GproteinDb are other notable new arrivals. Updates from DisProt, FuzDB and ELM comprehensively cover disordered proteins. Under the metabolism and signalling section Reactome, ConsensusPathDB, HMDB and CAZy are major returning resources. In microbial and viral genomes taxonomy and systematics are well covered by LPSN, TYGS and GTDB. Genomics resources include Ensembl, Ensembl Genomes and UCSC Genome Browser. Major returning pharmacology resource names include the IUPHAR/BPS guide and the Therapeutic Target Database. New plant databases include PlantGSAD for gene lists and qPTMplants for post-translational modifications. The entire Database Issue is freely available online on the Nucleic Acids Research website (https://academic.oup.com/nar). Our latest update to the NAR online Molecular Biology Database Collection brings the total number of entries to 1645. Following last year's major cleanup, we have updated 317 entries, listing 89 new resources and trimming 80 discontinued URLs. The current release is available at http://www.oxfordjournals.org/nar/database/c/.
© The Author(s) 2022. Published by Oxford University Press on behalf of Nucleic Acids Research.
PubMed Disclaimer
Similar articles
- The 2024 Nucleic Acids Research database issue and the online molecular biology database collection. Rigden DJ, Fernández XM. Rigden DJ, et al. Nucleic Acids Res. 2024 Jan 5;52(D1):D1-D9. doi: 10.1093/nar/gkad1173. Nucleic Acids Res. 2024. PMID: 38035367 Free PMC article.
- The 2023 Nucleic Acids Research Database Issue and the online molecular biology database collection. Rigden DJ, Fernández XM. Rigden DJ, et al. Nucleic Acids Res. 2023 Jan 6;51(D1):D1-D8. doi: 10.1093/nar/gkac1186. Nucleic Acids Res. 2023. PMID: 36624667 Free PMC article.
- The 27th annual Nucleic Acids Research database issue and molecular biology database collection. Rigden DJ, Fernández XM. Rigden DJ, et al. Nucleic Acids Res. 2020 Jan 8;48(D1):D1-D8. doi: 10.1093/nar/gkz1161. Nucleic Acids Res. 2020. PMID: 31906604 Free PMC article. Review.
- The 2018 Nucleic Acids Research database issue and the online molecular biology database collection. Rigden DJ, Fernández XM. Rigden DJ, et al. Nucleic Acids Res. 2018 Jan 4;46(D1):D1-D7. doi: 10.1093/nar/gkx1235. Nucleic Acids Res. 2018. PMID: 29316735 Free PMC article.
- The importance of biological databases in biological discovery. Baxevanis AD. Baxevanis AD. Curr Protoc Bioinformatics. 2011 Jun;Chapter 1:1.1.1-1.1.6. doi: 10.1002/0471250953.bi0101s34. Curr Protoc Bioinformatics. 2011. PMID: 21633941 Review.
- Beyond blast: enabling microbiologists to better extract literature, taxonomic distributions and gene neighbourhood information for protein families. Reed CJ, Denise R, Hourihan J, Babor J, Jaroch M, Martinelli M, Hutinet G, de Crécy-Lagard V. Reed CJ, et al. Microb Genom. 2024 Feb;10(2):001183. doi: 10.1099/mgen.0.001183. Microb Genom. 2024. PMID: 38323604 Free PMC article.
- Statistical integration of multi-omics and drug screening data from cell lines. El Bouhaddani S, Höllerhage M, Uh HW, Moebius C, Bickle M, Höglinger G, Houwing-Duistermaat J. El Bouhaddani S, et al. PLoS Comput Biol. 2024 Jan 31;20(1):e1011809. doi: 10.1371/journal.pcbi.1011809. eCollection 2024 Jan. PLoS Comput Biol. 2024. PMID: 38295113 Free PMC article.
- Ten simple rules for managing laboratory information. Berezin CT, Aguilera LU, Billerbeck S, Bourne PE, Densmore D, Freemont P, Gorochowski TE, Hernandez SI, Hillson NJ, King CR, Köpke M, Ma S, Miller KM, Moon TS, Moore JH, Munsky B, Myers CJ, Nicholas DA, Peccoud SJ, Zhou W, Peccoud J. Berezin CT, et al. PLoS Comput Biol. 2023 Dec 7;19(12):e1011652. doi: 10.1371/journal.pcbi.1011652. eCollection 2023 Dec. PLoS Comput Biol. 2023. PMID: 38060459 Free PMC article.
- The consequences of data dispersion in genomics: a comparative analysis of data sources for precision medicine. Costa M, García S A, Pastor O. Costa M, et al. BMC Med Inform Decis Mak. 2023 Nov 9;23(Suppl 3):256. doi: 10.1186/s12911-023-02342-w. BMC Med Inform Decis Mak. 2023. PMID: 37946154 Free PMC article.
- Immune Activation and Inflammatory Response Mediated by the NOD/Toll-like Receptor Signaling Pathway-The Potential Mechanism of Bullfrog ( Lithobates catesbeiana ) Meningitis Caused by Elizabethkingia miricola . Li F, Chen B, Xu M, Feng Y, Deng Y, Huang X, Geng Y, Ouyang P, Chen D. Li F, et al. Int J Mol Sci. 2023 Sep 26;24(19):14554. doi: 10.3390/ijms241914554. Int J Mol Sci. 2023. PMID: 37833994 Free PMC article.
- Cantelli G., Bateman A., Brooksbank C., Petrov A.I., Malik-Sheriff R.S., Ide-Smith M., Hermjakob H., Flicek P., Apweiler R., Birney E.et al. .. The European Bioinformatics Institute (EMBL-EBI) in 2021. Nucleic Acids Res. 2021; 10.1093/nar/gkab1127. - DOI - PMC - PubMed
- Sayers E.W., Bolton E.E., Brister J.R., Canese K., Chan J., Comeau D.C., Connor R., Funk K., Kelly C., Kim S.et al. .. Database resources of the national center for biotechnology information. Nucleic Acids Res. 2021; 10.1093/nar/gkab1112. - DOI - PMC - PubMed
- CNCB-NGDC Members and Partners Database Resources of the National Genomics Data Center, China National Center for Bioinformation in 2022. Nucleic Acids Res. 2021; 10.1093/nar/gkab951. - DOI - PMC - PubMed
- Torrens-Fontanals M., Peralta-García A., Talarico C., Guixà-González R., Giorgino T., Selent J.. SCoV2-MD: a database for the dynamics of the SARS-CoV-2 proteome and variant impact predictions. Nucleic Acids Res. 2021; 10.1093/nar/gkab977. - DOI - PMC - PubMed
- De Silva N.H., Bhai J., Chakiachvili M., Contreras-Moreira B., Cummins C., Frankish A., Gall A., Genez T., Howe K.L., Hunt S.E.et al. .. The Ensembl COVID-19 resource: ongoing integration of public SARS-CoV-2 data. Nucleic Acids Res. 2021; 10.1093/nar/gkab889. - DOI - PMC - PubMed
Publication types
- Search in MeSH
Related information
Grants and funding.
- Oxford University Press
LinkOut - more resources
Full text sources.
- Europe PubMed Central
- PubMed Central
- Silverchair Information Systems
Miscellaneous
- NCI CPTAC Assay Portal
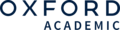
- Citation Manager
NCBI Literature Resources
MeSH PMC Bookshelf Disclaimer
The PubMed wordmark and PubMed logo are registered trademarks of the U.S. Department of Health and Human Services (HHS). Unauthorized use of these marks is strictly prohibited.
- Search Menu
- Sign in through your institution
- Chemical Biology and Nucleic Acid Chemistry
- Computational Biology
- Critical Reviews and Perspectives
- Data Resources and Analyses
- Gene Regulation, Chromatin and Epigenetics
- Genome Integrity, Repair and Replication
- Methods Online
- Molecular Biology
- Nucleic Acid Enzymes
- RNA and RNA-protein complexes
- Structural Biology
- Synthetic Biology and Bioengineering
- Advance Articles
- Breakthrough Articles
- Special Collections
- Scope and Criteria for Consideration
- Author Guidelines
- Data Deposition Policy
- Database Issue Guidelines
- Web Server Issue Guidelines
- Submission Site
- About Nucleic Acids Research
- Editors & Editorial Board
- Information of Referees
- Self-Archiving Policy
- Dispatch Dates
- Advertising and Corporate Services
- Journals Career Network
- Journals on Oxford Academic
- Books on Oxford Academic
Initial Submission Online
Prepare your manuscript as detailed here and then submit online via the web site .
CHECKLIST prior to initial submission:
You must ensure that you have available the following files. Acceptable file formats are listed here . Please ensure that all files are named carefully and unambiguously so that their content is clear. For original submissions, a single PDF including main text, figures and tables is the preferred format. Supplementary file(s) should be uploaded separately.
- A PDF file of your complete manuscript text including tables, figures and their captions.
- Any supplementary data MUST be in a file(s) separate from the main manuscript file
- A file of any related manuscript currently under consideration by another journal (see Publication Ethics )
- Your manuscript title and abstract text for cutting and pasting into the system
- The email addresses of all of your co-authors [Please note that the journal reserves the right to contact the Senior Author of the manuscript if his/her contact details are not included.]
- Names, institutes and email addresses of at least six suggested Referees. They should be scientists working independently (i.e. not a recent collaborator) in areas similar to your own who have relevant expertise, such as those included in your reference list. If you have any queries please contact the Editorial Office ( [email protected] ).
- Your letter to the Editor. The letter must contain details of any previous submissions of the work to NAR (see Publication Ethics )
- Between two and five keywords or short relevant phrases
Browser compatibility
The following browsers are compatible with the NAR online submission system: Internet Explorer 9, 10, 11, Firefox 32, Chrome 37, Safari 6, 7. For more details click 'System requirements' on the log-in screen.
Submitting your manuscript
- If you already have a user account (i.e. you have submitted or reviewed a manuscript on this system before) use your existing User ID and Password. (Your user ID may be your email address.)
- If you do not know your login details, check to see if you are already registered by entering your email address into the 'Password Help' box. If your email address has changed recently please check that there is no account under a previous email address. If you are not already registered, you can register by clicking on the 'Create account' button at the top right of the Log In screen and following the on-screen instructions.
- If you have trouble finding manuscripts, or other problems with your account, do not create another account. Instead, please contact the Editorial Office ( [email protected] ).
- NAR supports the ORCID initiative. All submitting authors are required to register with ORCID and link their ORCID ID to their ScholarOne account. For more information on ORCID and how to register or link accounts, please visit this page .
- To submit a new manuscript, go to your 'Author Centre', click on 'Start new submission', and then follow the on-screen instructions. There are 7 steps to follow to submit your manuscript and you can monitor progress from the checklist on the left. You move from one step to the next by clicking on the 'Save and Continue' button on each screen. Please note that if you click on the 'Back' or 'Forward' buttons on your browser, the information you have entered will not be saved. Enter your manuscript data into the relevant fields, following the instructions at the top of each screen. It is compulsory to complete many of the fields, which are marked 'req'. At any stage you can stop the submission process by clicking on the 'Main Menu' button. Everything you have typed into the system will be saved, and the partially completed submission will appear under 'Unsubmitted and Manuscripts in Draft' in your 'Author Centre'. To return to the submission process you will need to click on the button 'Continue' against the relevant manuscript title. Files and metadata will be saved in the system for 30 days from the start of your submission process, so it is essential that you complete submission within this time.
- Locate individual files using the 'Browse' buttons and select the appropriate 'File type' from the pull-down menu. One of the files must be a 'Manuscript File'.
- Upload your files (in groups of up to five) by clicking on the 'Upload files' button. This may take several minutes. A number of screens (one per file) will appear, in which you can provide figure/table captions. Click 'save' to confirm the upload of the file.
- If you have more than five files to upload you should repeat this procedure until all are uploaded.
- Indicate the order in which the files should appear. This is particularly important for figure files as it will determine the order in which they appear in the consolidated PDF used for peer review.
- After your files have been uploaded, you should view and proofread your manuscript, by clicking on the PDF button and the HTML button. If the files have not been uploaded to your satisfaction, go back to the file upload screen where you can remove the files you do not want and repeat the process.
- When you are satisfied with the uploaded manuscript proof click on 'Save and Continue' which will take you to the 'Review & Submit' screen. The system will check that you have completed all the mandatory fields and that you have viewed your manuscript proof. It will also present you with a summary of all the information you have provided and give you a final chance to edit it. If there is a red cross next to any section this will indicate that not all the fields have been filled in correctly. You may either go back to the relevant page or click the nearest 'edit' button.
- When you have finished reviewing this information press 'Submit'. You should do this within 30 days of the start of your submission process to avoid losing your data.
- After the manuscript has been submitted you will see a confirmation screen and receive an email confirmation stating that your manuscript has been successfully submitted. This will also give the assigned manuscript number, which is used in all correspondence during peer review. If you do not receive this, your manuscript will not have been successfully submitted to the journal and the paper cannot progress to peer review. If this is the case your manuscript will still be sitting in the 'Unsubmitted Manuscripts' section of your 'Author Centre' awaiting your attention.
- If you return to your 'Author Centre' you will notice that your newly submitted manuscript can be found in the 'Submitted Manuscripts' area. The 'Status' section provides information on the status of your manuscript as it moves through the review process.
- After you have completed the submission process, if you then discover a mistake (e.g. missing or incorrect figures), do NOT go through the submission process a second time. Instead, please contact the Editorial Office ( [email protected] ) for advice.
Uploading your revised manuscript
- Log on to the online submission web site as before and, in the 'Author Centre', click on the purple button 'Manuscripts Awaiting Revision'. You will see those manuscripts that require a revision (or that have been revised). Locate the correct manuscript and create a revision by clicking on 'Create a Revision' under Actions. You will be able to see the Editor and reviewer comments and to respond to these.
- The 'Upload files' screen will automatically be populated with the files that you uploaded at initial submission. You should delete all files that have been changed during revision and upload your revised files in their place, by the procedure used during initial submission. You will also be able to amend, if necessary, any of the associated manuscript information, using the same 7 steps followed in your initial submission. If you wish to complete the process another time, you will find the manuscript in your 'Revised manuscripts in draft' list.
A editable file of your complete manuscript including title page and abstract with changed text marked in red. The file should be in .doc, .rtf, or LaTeX format NOT .pdf.
Your high-resolution figure files. Detailed guidelines are available . Please make sure that each figure is clearly labelled with its number.
Complex tables should be uploaded separately. Detailed guidelines are available . Please make sure that each table is clearly labelled with its number.
Any supplementary data MUST be in a file(s) separate from the main manuscript file.
- If you click on 'View comments/respond' you will see the Editor's letter to you together with the Referees' comments. You must cut and paste your responses into the text areas at the bottom of the screen. If you wish to upload any supplementary information not intended for publication, you should upload it as a 'cover letter' file.
- When you have completed your revision, press the 'Submit' button. If your revised manuscript has been successfully submitted, you will see a confirmation screen showing your manuscript number; this will be the same as that of your initial submission with the prefix 'R1' (or R2, R3 as appropriate). You will also receive an email confirming the submission.
- Editorial Board
Affiliations
- Online ISSN 1362-4962
- Print ISSN 0305-1048
- Copyright © 2024 Oxford University Press
- About Oxford Academic
- Publish journals with us
- University press partners
- What we publish
- New features
- Open access
- Institutional account management
- Rights and permissions
- Get help with access
- Accessibility
- Advertising
- Media enquiries
- Oxford University Press
- Oxford Languages
- University of Oxford
Oxford University Press is a department of the University of Oxford. It furthers the University's objective of excellence in research, scholarship, and education by publishing worldwide
- Copyright © 2024 Oxford University Press
- Cookie settings
- Cookie policy
- Privacy policy
- Legal notice
This Feature Is Available To Subscribers Only
Sign In or Create an Account
This PDF is available to Subscribers Only
For full access to this pdf, sign in to an existing account, or purchase an annual subscription.

Organic Chemistry Frontiers
Site-specific dna post-synthetic modification via fast photocatalytic allylation.
Developing new chemical toolboxes for site-specific nucleic acid post-synthetic modification is of great challenge and urgently required for precisely expanding DNA functionality, which holds significant research implications in nucleic acid chemistry, biology, and beyond. Herein, we demonstrate the first site-specific DNA post-synthetic modification via fast photocatalytic allylation under visible light. Allyl sulfone groups were introduced into oligonucleotides, not only at the terminal sites via traditional amidation reactions but also at the internal and terminal sites via DNA solid-phase synthesis. This visible-light-induced photocatalytic decarboxylative allylation proceeds rapidly on the oligonucleotides bearing allyl sulfone groups under open-to-air conditions within minutes, exhibiting excellent chemoselectivity and compatibility with various functional groups while retaining the DNA integrity. Specifically, introducing allyl sulfone into oligonucleotides via DNA solid-phase synthesis enables site-specific DNA modification on chemically synthesized single-stranded DNA at internal and terminal positions, hybridized double-stranded DNA, and enzymatically amplified long-chain DNA under visible light. The versatile reactivity of allyl sulfone scaffolds further enables diverse on-DNA photocatalytic transformations, promising to advance chemical toolboxes for DNA post-synthetic modification through diverse photochemical methods.
Supplementary files
- Supplementary information PDF (5094K)
Article information
Download citation, permissions.

Y. Huang, Y. Zhang, C. Hu and Y. Chen, Org. Chem. Front. , 2024, Accepted Manuscript , DOI: 10.1039/D4QO00752B
To request permission to reproduce material from this article, please go to the Copyright Clearance Center request page .
If you are an author contributing to an RSC publication, you do not need to request permission provided correct acknowledgement is given.
If you are the author of this article, you do not need to request permission to reproduce figures and diagrams provided correct acknowledgement is given. If you want to reproduce the whole article in a third-party publication (excluding your thesis/dissertation for which permission is not required) please go to the Copyright Clearance Center request page .
Read more about how to correctly acknowledge RSC content .
Social activity
Search articles by author.
This article has not yet been cited.
Advertisements

IMAGES
VIDEO
COMMENTS
Nucleic Acids Research: 50 years of research for scientists by scientists . NAR's 50 years of forming relationships with our author community has allowed the journal to continue paving the way with cutting-edge, reputable research in the nucleic acids and protein fields. View our information for authors
About the journal. Nucleic Acids Research ( NAR) publishes the results of leading-edge research into physical, chemical, biochemical and biological aspects of nucleic acids and proteins involved in nucleic acid metabolism and/or interactions. It enables the rapid publication of papers under the following categories: Chemistry and Nucleic Acid ...
Publishes the results of leading edge research into physical, chemical, biochemical and biological aspects of nucleic acids and proteins involved in nucleic acid metabolism and/or interactions. Fully open access.
Nucleic Acids Research is an open-access peer-reviewed scientific journal published since 1974 by the Oxford University Press. The journal covers research on nucleic acids, such as DNA and RNA, and related work. According to the Journal Citation Reports, the journal's 2021 impact factor is 19.160.
Read the latest Research articles in Nucleic acids from Nature. ... View all journals; Search; My Account Login; ... arabino nucleic acids, 2′-fluoroarabino nucleic acids, hexitol nucleic acids ...
A high-resolution map of functional miR-181 response elements in the thymus reveals the role of coding sequence targeting and an alternative seed match
Nucleic acids (DNA or RNA) are polymers of nucleotides - each nucleotide consists of a pentose sugar, a phosphate group and one of the nitrogenous bases (purines and pyrimidines). Nucleic acids ...
Here, Bou-Nader et al., define the nucleic acid-binding specificity of S9.6 and report its crystal structures free and bound to a hybrid, which reveal the asymmetric recognition of the RNA and DNA ...
Nucleic acid chemistry. Since the discovery of the double-helical structure of DNA and postulation of the central dogma of molecular biology, stating that the flow of genetic information goes from ...
Nucleic Acids Research NAR. 0305-1048 (Print) / 1362-4962 (Online) Website ISSN Portal About Articles About. Publishing with this journal. The journal charges up to: 3802 USD; as ... This journal began publishing in open access in 2005.
Nucleic Acids Research is a peer reviewed fully open access journal publishing 24 issues per year online and in print. All papers published in the Journal are made freely available online under open access publishing agreements, with applicable charges. ... Ethical research. The Journal follows Committee on Publication Ethics (COPE) guidelines ...
Learn about the journal profile, impact factor, ranking, and citation analysis of Nucleic Acids Research, a leading journal in the Web of Science Core Collection.
Publishes the results of leading edge research into physical, chemical, biochemical and biological aspects of nucleic acids and proteins involved in nucleic acid metabolism and/or interactions. Fully open access.
Journal List; Nucleic Acids Res; Nucleic Acids Research Vols. 1 to 52; 1974 to 2024; Vol. 52 2024: v.52(D1): D1-D1702 2024 Jan 5: v.52(1): 1-509 2024 Jan 11: ... Articles from Nucleic Acids Research are provided here courtesy of Oxford University Press. Follow NCBI. Connect with NLM National Library of Medicine 8600 Rockville Pike ...
Scope. NAR provides rapid publication of papers on physical, chemical, biochemical and biological aspects of nucleic acids and proteins involved in nucleic acid metabolism and/or interactions. All manuscripts must present some novel development and meet the general criteria of originality, timeliness, significance and scientific excellence.
Abstract. The 2021 Nucleic Acids Research database Issue contains 189 papers spanning a wide range of biological fields and investigation. It includes 89 papers reporting on new databases and 90 covering recent changes to resources previously published in the Issue. A further ten are updates on databases most recently published elsewhere.
NAR Journals. Issues Section browse Chemical Biology and Nucleic Acid Chemistry ... The 22nd annual Nucleic Acids Research Web Server Issue 2024 . Editorial: Gary Felsenfeld (1929-2024) Advertisement. close advertisement. Advertisement. About Nucleic Acids Research; Editorial Board; Policies; Author Guidelines;
Abstract. Nucleic acids, deoxyribonucleic acid (DNA) and ribonucleic acid (RNA), carry genetic information which is read in cells to make the RNA and proteins by which living things function. The well-known structure of the DNA double helix allows this information to be copied and passed on to the next generation.
The 2022 Nucleic Acids Research Database Issue contains 185 papers, including 87 papers reporting on new databases and 85 updates from resources previously published in the Issue. Thirteen additional manuscripts provide updates on databases most recently published elsewhere. Seven new databases focus specifically on COVID-19 and SARS-CoV-2 ...
Nucleic Acids Research (NAR) publishes the results of leading-edge research into physical, chemical, biochemical and biological aspects of nucleic acids a
Journal overview. Nucleosides, Nucleotides & Nucleic Acids publishes research articles, short notices, and concise, critical reviews of related topics that focus on the chemistry and biology of nucleosides, nucleotides, and nucleic acids. Complete with experimental details, this all-inclusive journal emphasizes the synthesis, biological ...
Journal of Nucleic Acids is a peer-reviewed, Open Access journal that publishes original research articles as well as review articles covering all structural, chemical, and functional aspects of DNA and RNA research.. Although not limited to merely these areas, some fields of interest include DNA and RNA research that relate to cancer and other diseases, various interplay of the biological ...
The rapid decline in DNA sequencing costs has fueled the demand for nucleic acid collection to unravel genomic information, develop treatments for genetic diseases, and track emerging biological threats. Current approaches to maintaining these nucleic acid collections hinge on continuous electricity for maintaining low-temperature and intricate cold-chain logistics. Inspired by the millennia ...
Nucleic Acids Research has an h-index of 607.It means 607 articles of this journal have more than 607 number of citations. The h-index is a way of measuring the productivity and citation impact of the publications. The h-index is defined as the maximum value of h such that the given journal/author has published h papers that have each been cited at least h number of times.
A file of any related manuscript currently under consideration by another journal (see Publication Ethics) ... The 22nd annual Nucleic Acids Research Web Server Issue 2024 . Editorial: Gary Felsenfeld (1929-2024) Advertisement. Advertisement. close advertisement. Advertisement.
Developing new chemical toolboxes for site-specific nucleic acid post-synthetic modification is of great challenge and urgently required for precisely expanding DNA functionality, which holds significant research implications in nucleic acid chemistry, biology, and beyond. Herein, we demonstrate the first si ... From the journal: